Chapter 26 1. Differentiate among the structures and functions of the upper respiratory tract, the lower respiratory tract, and the chest wall. 2. Describe the process that initiates and controls inspiration and expiration. 3. Describe the process of gas diffusion within the lungs. 4. Identify the respiratory defense mechanisms. 5. Describe the significance of arterial blood gas values in relation to respiratory function. 6. Relate the signs and symptoms of inadequate oxygenation to implications of these findings. 7. Link the age-related changes of the respiratory system to the differences in assessment findings. 8. Select the significant subjective and objective data related to the respiratory system that should be obtained from a patient. 9. Select appropriate techniques to use in the physical assessment of the respiratory system. 10. Differentiate normal from common abnormal findings in a physical assessment of the respiratory system. 11. Describe the purpose, significance of results, and nursing responsibilities related to diagnostic studies of the respiratory system. The primary purpose of the respiratory system is gas exchange. This involves the transfer of oxygen (O2) and carbon dioxide (CO2) between the atmosphere and the blood. The respiratory system is divided into two parts: the upper respiratory tract and the lower respiratory tract (Fig. 26-1). eTABLE 26-1 OXYGEN-HEMOGLOBIN DISSOCIATION CURVE Air moves through the oropharynx to the laryngopharynx. It then travels through the epiglottis to the larynx before moving into the trachea. The epiglottis is a small flap located behind the tongue that closes over the larynx during swallowing. This prevents solids and liquids from entering the lungs. The vocal cords are located in the larynx. Vibrational sounds are made during respiration leading to vocalization. Air passes through the glottis, the opening between the vocal cords, and into the trachea. The trachea is a cylindric tube about 5 in (10 to 12 cm) long and 1 in (1.5 to 2.5 cm) in diameter. U-shaped cartilages keep the trachea open but allow the adjacent esophagus to expand for swallowing. The trachea bifurcates into the right and left mainstem bronchi at a point called the carina. The carina is located at the level of the manubriosternal junction, also called the angle of Louis. The carina is highly sensitive, and touching it during suctioning causes vigorous coughing.1 The lower respiratory tract consists of the bronchi, bronchioles, alveolar ducts, and alveoli. With the exception of the right and left mainstem bronchi, all lower airway structures are located inside the lungs. The right lung is divided into three lobes (upper, middle, and lower) and the left lung into two lobes (upper and lower) (Fig. 26-2). The structures of the chest wall (ribs, pleura, muscles of respiration) are also important for respiration. The mainstem bronchi subdivide several times to form the lobar, segmental, and subsegmental bronchi. Further divisions form the bronchioles. The most distant bronchioles are called the respiratory bronchioles. Beyond these lie the alveolar ducts and alveolar sacs (Fig. 26-3). The bronchioles are encircled by smooth muscles that constrict and dilate in response to various stimuli. The terms bronchoconstriction and bronchodilation refer to a decrease or increase in the diameter of the airways caused by contraction or relaxation of these muscles. After moving through the Vd, air reaches the respiratory bronchioles and alveoli (Fig. 26-4). Alveoli are small sacs that are the primary site of gas exchange in the lungs. The alveoli are interconnected by pores of Kohn, which allow movement of air from alveolus to alveolus (see Fig. 26-1). Deep breathing promotes air movement through these pores and assists in moving mucus out of the respiratory bronchioles. Bacteria can also move through these pores, spreading infection to previously uninfected areas. The adult lung has 300 million alveoli. Alveoli have a total volume of about 2500 mL and a surface area for gas exchange that is about the size of a tennis court. Gases are exchanged at the alveolar-capillary membrane where the alveoli come in contact with pulmonary capillaries (Fig. 26-5). In conditions such as pulmonary edema, excess fluid fills the interstitial space and alveoli, markedly reducing gas exchange. When not enough surfactant is present, the alveoli collapse. The term atelectasis refers to collapsed, airless alveoli (see Fig. 26-4, B). The postoperative patient is at risk for atelectasis because of the effects of anesthesia and restricted breathing with pain (see Chapter 20). In acute respiratory distress syndrome (ARDS), lack of surfactant contributes to widespread atelectasis (see Chapter 68). Ventilation involves inspiration, or inhalation (movement of air into the lungs), and expiration, or exhalation (movement of air out of the lungs). Air moves in and out of the lungs because intrathoracic pressure changes in relation to pressure at the airway opening. Contraction of the diaphragm and intercostal and scalene muscles increases chest dimensions, thereby decreasing intrathoracic pressure. Gas flows from an area of higher pressure (atmospheric) to one of lower pressure (intrathoracic).2 When dyspnea (shortness of breath) occurs, neck and shoulder muscles can assist the effort. Some conditions (e.g., phrenic nerve paralysis, rib fractures, neuromuscular disease) may limit diaphragm or chest wall movement and cause the patient to breathe with smaller tidal volumes. As a result, the lungs do not fully inflate, and gas exchange is impaired. Exacerbations of asthma or chronic obstructive pulmonary disease (COPD) cause expiration to become an active, labored process (see Chapter 29). Abdominal, intercostal, and accessory muscles (e.g., scalene, trapezius) assist in expelling air during labored breathing. Two methods are used to assess the efficiency of gas transfer in the lung and tissue oxygenation: analysis of arterial blood gases (ABGs) and pulse oximetry. ABGs are measured to determine oxygenation status and acid-base balance. ABG analysis includes measurement of the PaO2, PaCO2, acidity (pH), and bicarbonate (HCO3−) in arterial blood. The SaO2 is either calculated or measured during this analysis. Normal values for ABGs are given in Table 26-1. TABLE 26-1 NORMAL ARTERIAL AND VENOUS BLOOD GAS VALUES* *Assumes patient is ≤60 yr of age and breathing room air. ‡The same normal values apply when SpO2 and SvO2 are obtained by oximetry. The normal PaO2 decreases with advancing age. It also varies in relation to the distance above sea level. At higher altitudes the barometric pressure is lower, resulting in a lower inspired oxygen pressure and a lower PaO2 (see Table 26-1). For the patient with a normal or near-normal cardiac status, an assessment of PaO2 or SaO2 is usually sufficient to determine the level of oxygenation. The patient with impaired cardiac output or hemodynamic instability may have inadequate tissue oxygen delivery or abnormal oxygen consumption.3 The amount of oxygen delivered to the tissues or consumed can be calculated. A catheter positioned in the pulmonary artery, termed a pulmonary artery (PA) catheter, is used for mixed venous sampling (see Chapter 66). Blood drawn from a PA catheter is termed a mixed venous blood gas sample because it consists of venous blood that has returned to the heart and “mixes” in the right ventricle. Normal mixed venous values are given in Table 26-1. When tissue oxygen delivery is inadequate or when inadequate oxygen is transported to the tissues by the hemoglobin, the PvO2 and SvO2 fall. Arterial oxygen saturation can be monitored noninvasively and continuously using a pulse oximetry probe on the finger, toe, ear, or bridge of the nose4 (see eFig. 26-2 available on the website). The abbreviation SpO2 is used to indicate the oxygen saturation of hemoglobin as measured by pulse oximetry. SpO2 and heart rate are displayed on the monitor as digital readings (see eFig. 26-2). Pulse oximetry is particularly valuable in intensive care and perioperative situations, in which sedation or decreased consciousness might mask hypoxia (Table 26-2). SpO2 is assessed with each routine vital sign check in many inpatient areas. Changes in SpO2 can be detected quickly and treated (Table 26-3). Oximetry is also used during exercise testing and when adjusting flow rates during long-term oxygen therapy. TABLE 26-2 MANIFESTATIONS OF INADEQUATE OXYGENATION TABLE 26-3 CRITICAL VALUES FOR PaO2 AND SpO2* *The same critical values apply for SpO2 and SaO2. Values pertain to rest or exertion. In a healthy person an increase in PaCO2 or a decrease in pH causes an immediate increase in the respiratory rate. The PaCO2 does not vary more than about 3 mm Hg if lung function is normal. Conditions such as COPD alter lung function and may result in chronically elevated PaCO2 levels. In these instances the patient is relatively insensitive to further increases in PaCO2 as a stimulus to breathe and may be maintaining ventilation largely because of a hypoxic drive from the peripheral chemoreceptors (see Chapter 29). The velocity of airflow slows greatly after it passes the larynx, facilitating the deposition of smaller particles (1 to 5 µm). They settle out the way sand does in a river, a process termed sedimentation. Particles less than 1 µm in size are too small to settle in this manner and are deposited in the alveoli. An example of small particles that can build up is coal dust, which can lead to pneumoconiosis (see Chapter 28). Below the larynx, the movement of mucus is accomplished by the mucociliary clearance system, commonly referred to as the mucociliary escalator. This term is used to indicate the relationship between the secretion of mucus and the ciliary activity. Mucus is continuously secreted at a rate of about 100 mL/day by goblet cells and submucosal glands. It forms a mucous blanket that contains the impacted particles and debris from distal lung areas (see Fig. 26-1). The small amount of mucus normally secreted is swallowed without being noticed. Secretory immunoglobulin A (IgA) in the mucus helps protect against bacteria and viruses. Cilia cover the airways from the level of the trachea to the respiratory bronchioles (see Fig. 26-1). Each ciliated cell contains approximately 200 cilia, which beat rhythmically about 1000 times per minute in the large airways, moving mucus toward the mouth. The ciliary beat is slower further down the tracheobronchial tree. As a consequence, particles that penetrate more deeply into the airways are removed less rapidly. Ciliary action is impaired by dehydration; smoking; inhalation of high oxygen concentrations; infection; and ingestion of drugs such as atropine, anesthetics, alcohol, or cocaine. Patients with COPD and cystic fibrosis have repeated lower respiratory tract infections. Cilia are often destroyed during these infections, resulting in impaired secretion clearance; a chronic productive cough; and chronic colonization by bacteria, which leads to frequent respiratory tract infections. Because ciliated cells are not found below the level of the respiratory bronchioles, the primary defense mechanism at the alveolar level is alveolar macrophages. Alveolar macrophages rapidly phagocytize inhaled foreign particles such as bacteria. The debris is moved to the level of the bronchioles for removal by the cilia or removed from the lungs by the lymphatic system. Particles (e.g., coal dust, silica) that cannot be adequately phagocytized tend to remain in the lungs for indefinite periods and can stimulate inflammatory responses (see Chapter 28). Because alveolar macrophage activity is impaired by cigarette smoke, the smoker who is employed in an occupation with heavy dust exposure (e.g., mining, foundries) is at an especially high risk for lung disease. Age-related changes in the respiratory system can be divided into alterations in structure, defense mechanisms, and respiratory control (Table 26-4). Structural changes include calcification of the costal cartilages, which can interfere with chest expansion. The outward curvature of the spine is marked, especially with osteoporosis, and the lumbar curve flattens. Therefore the chest may appear barrel shaped, and the older person may need to use accessory muscles to breathe. Respiratory muscle strength progressively declines after age 50. Overall, the lungs in the older adult are harder to inflate.5 TABLE 26-4 GERONTOLOGIC ASSESSMENT DIFFERENCES Greater ↓ in PaO2 and ↑ in PaCO2 before respiratory rate changes ↓ Ability to maintain acid-base balance Significant hypoxemia or hypercapnia may develop from relatively small incidents Retained secretions, excessive sedation, or positioning that impairs chest expansion may substantially alter PaO2 or SpO2 values Many older adults lose subcutaneous fat, and bony prominences are pronounced. Within the lung, the number of functional alveoli decreases, and they become less elastic. Small airways in the lung bases close earlier in expiration. As a consequence, more inspired air is distributed to the lung apices and ventilation is less well matched to perfusion, lowering the PaO2. Therefore older adults have less tolerance for exertion, and dyspnea can occur if their activity exceeds their normal exercise.6
Nursing Assessment
Respiratory System
Structures and Functions of Respiratory System
Upper Respiratory Tract
Lower Respiratory Tract
Surfactant.
Physiology of Respiration
Ventilation.
Arterial Blood Gases.
Arterial Blood Gases
Venous Blood Gases
Laboratory Value
Sea Level BP 760 mm Hg
1 Mile Above Sea Level BP 629 mm Hg
Mixed Venous Blood Gases
pH
7.35-7.45
7.35-7.45
pH
7.32-7.43
PaO2†
80-100 mm Hg
65-75 mm Hg
PvO2
38-42 mm Hg
SaO2†
>95%‡
>95%‡
SvO2
60%-80%‡
PaCO2
35-45 mm Hg
35-45 mm Hg
PvCO2
38-55 mm Hg
HCO3−
22-26 mEq/L (mmol/L)
22-26 mEq/L (mmol/L)
HCO3−
22-26 mEq/L (mmol/L)
Mixed Venous Blood Gases.
Oximetry.
Onset
Manifestations
Early
Late
Central Nervous System
Unexplained apprehension
X
Unexplained restlessness or irritability
X
Unexplained confusion or lethargy
X
X
Combativeness
X
Coma
X
Respiratory
Tachypnea
X
Dyspnea on exertion
X
Dyspnea at rest
X
Use of accessory muscles
X
Retraction of interspaces on inspiration
X
Pause for breath between sentences, words
X
Cardiovascular
Tachycardia
X
Mild hypertension
X
Dysrhythmias
X
X
Hypotension
X
Cyanosis
X
Cool, clammy skin
X
Other
Diaphoresis
X
X
Decreased urine output
X
X
Unexplained fatigue
X
X
PaO2 (%)
SpO2 (%)
Significance
≥70
≥94
Adequate unless patient is hemodynamically unstable or hemoglobin (Hgb) has difficulty releasing O2 to the tissues.
60
90
Adequate in almost all patients. Provides adequate oxygenation but with less margin for error than above.
55
88
Adequate for patients with chronic hypoxemia if no cardiac problems occur. These values are also used as criteria for prescription of continuous O2 therapy.
40
75
Inadequate but may be acceptable on a short-term basis if the patient also has CO2 retention. In this situation, respirations may be stimulated by a low PaO2. Thus the PaO2 cannot be raised rapidly.
<40
<75
Inadequate. Tissue hypoxia and cardiac dysrhythmias can be expected.
Control of Respiration
Chemoreceptors.
Respiratory Defense Mechanisms
Filtration of Air.
Mucociliary Clearance System.
Alveolar Macrophages.
Gerontologic Considerations
Effects of Aging on Respiratory System
Respiratory System
Changes
Differences in Assessment Findings
Structures
Defense Mechanisms
Respiratory Control
Nursing Assessment: Respiratory System
Get Clinical Tree app for offline access
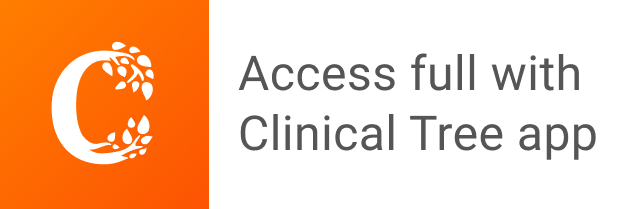