CHAPTER 8 Drug sensitivity varies with age. Infants are especially sensitive to drugs, as are the elderly. In the very young, heightened drug sensitivity is the result of organ immaturity. In the elderly, heightened sensitivity results largely from organ degeneration. Other factors that affect sensitivity in the elderly are increased severity of illness, the presence of multiple pathologies, and treatment with multiple drugs. The clinical challenge created by heightened drug sensitivity in the very young and in the elderly is the subject of Chapters 10 and 11, respectively. The impact of kidney disease is illustrated in Figure 8–1, which shows the decline in plasma levels of kanamycin (an antibiotic) following injection into two patients, one with healthy kidneys and one with renal failure. (Elimination of kanamycin is exclusively renal.) As indicated, kanamycin levels fall off rapidly in the patient with good kidney function. In this patient, the drug’s half-life is brief—only 1.5 hours. In contrast, drug levels decline very slowly in the patient with renal failure. Because of kidney disease, the half-life of kanamycin has increased by nearly 17-fold—from 1.5 hours to 25 hours. Under these conditions, if dosage is not reduced, kanamycin will quickly accumulate to dangerous levels. Until recently, the power of the placebo effect was unquestioned by most clinicians and researchers. However, evidence now suggests that responses to placebos may be much smaller than previously believed (Box 8–1).
Individual variation in drug responses
Age
Pathophysiology
Kidney disease
Effect of renal failure on kanamycin half-life.
Kanamycin was administered at time “0” to two patients, one with healthy kidneys and one with renal failure. Note that drug levels declined very rapidly in the patient with healthy kidneys and extremely slowly in the patient with renal failure, indicating that renal failure greatly reduced the capacity to remove this drug from the body. (T1/2 = half-life.)
Placebo effect
Stay updated, free articles. Join our Telegram channel
Full access? Get Clinical Tree
Individual variation in drug responses
Get Clinical Tree app for offline access
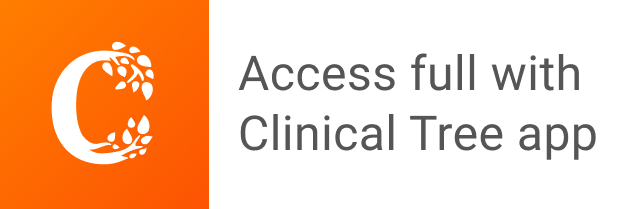