Genetics, Conception, and Fetal Development
On completion of this chapter, the reader will be able to:
• Explain the key concepts of basic human genetics.
• Explore how recent advances in genetics have changed the field of health care.
• Discuss key findings and the ethical, legal, and social implications of the Human Genome Project.
• Describe expanded roles for nurses in genetics and genetic counseling.
• Identify genetic disorders commonly tested for in maternity and newborn nursing.
• Discuss the current status of gene therapy.
• Summarize the process of fertilization.
• Describe the development, structure, and functions of the placenta.
• Describe the composition and functions of the amniotic fluid.
• Identify three organs or tissues arising from each of the three primary germ layers.
• Summarize the significant changes in growth and development of the embryo and fetus.
• Identify the potential effects of teratogens during vulnerable periods of embryonic and fetal development.
This chapter presents a brief discussion of genetics and the role of the nurse in genetics. It also provides an overview of the process of fertilization and of the development of the normal embryo and fetus.
Genetics
Recent advances in molecular biology and genomics have revolutionized the field of health care by providing the tools needed to determine the hereditary component of many diseases as well as improve our ability to predict susceptibility to disease, onset and progression of disease, and response to medications (Guttmacher, McGuire, Ponder, et al., 2010). This increase in genetic knowledge has resulted in a gradual shift from genetics to genomics. Genetics is the study of individual genes and their effect on relatively rare single-gene disorders, whereas genomics is the study of all the genes in the human genome together, including their interactions with each other, the environment, and the influence of other psychosocial factors and cultural factors. Genes are basic physical units of inheritance that are passed from parents to offspring and contain the information needed to specify traits. The genome is the entire set of genetic instructions found in each cell. For these and other definitions of genetic terms, visit the Talking Glossary of Genetic Terms (www.genome.gov/Glossary).
Genetic services are rapidly becoming an integral part of routine health care as a result of:
• Growing public interest in personalized genomic information (information about much or all of a person’s genome)
• Increasing development of practice guidelines
• Mounting commercial pressures
• Ever-increasing opportunities for individuals, families, and communities to participate in the direction and design of their genomic health care (Guttmacher, McGuire, Ponder, et al., 2010)
Moreover, many individuals and families have participated in direct-to-consumer genetic testing (testing marketed directly to consumers through television, print advertisements, and websites for companies such as DNA Direct [www.dnadirect.com/web], 23 and Me [www.23andme.com], and DeCODEme [www.decodeme.com]). Although much of the information provided by direct-to-consumer testing companies is recreational (e.g., ancestry information, information about type of ear wax, bitter taste perception), some of it is health related and could be interpreted as diagnosis (Evans and Green, 2009). Because of this, direct-to-consumer testing that is provided without the involvement of competent health care professionals may be not only unhelpful but even harmful (Guttmacher, McGuire, Ponder, et al., 2010; McGuire and Burke, 2010).
Nursing Expertise in Genetics and Genomics
Genetic disorders span every clinical practice specialty and site, including schools, clinics, offices, hospitals, mental health agencies, and community health settings. Because the potential impact on families and the community is significant, genetic information, technology, and testing must be incorporated into health care services. Genetics must be integrated into nursing education and practice.
Expanded roles for nurses with expertise in genetics and genomics are developing in many areas of maternity and women’s health nursing. These areas include but are not limited to:
• Preconception counseling and testing
• Neonatal genetic screening and testing
• Palliative care for infants with life-threatening genetic conditions and their families
• The identification and care of individuals with genetic conditions and their families
• The care of women with genetic conditions who require specialized care during pregnancy, such as women with congenital heart disease, cystic fibrosis, and factor V Leiden.
Essential Competencies in Genetics and Genomics for All Nurses
Nearly 50 organizations, including the Association of Women’s Health, Obstetric and Neonatal Nurses (AWHONN) and the National Association of Neonatal Nurses (NANN), have endorsed the Essentials of Genetic and Genomic Nursing: Competencies, Curricula Guidelines, and Outcome Indicators (ed 2) (Consensus Panel on Genetic/Genomic Nursing Competencies, 2009). The competencies in the document reflect the minimum amount of genetic and genomic competency expected of all nurses. The competencies are not intended to replace or recreate current standards of practice. The document is available at www.genome.gov/Pages/Careers/HealthProfessionalEducation/geneticscompetency.pdf. Some of the competencies most relevant to nurses in maternity nursing include:
• Constructs a pedigree from collected family history information using standardized symbols and terminology
• Develops a plan of care that incorporates genetic and genomic assessment information
• Provides patients with credible, accurate, appropriate, and current genetic and genomic information, resources, services, and/or technologies that facilitate decision making
• Recognizes when one’s own attitudes and values related to genetic and genomic science may affect care provided to patients
• Facilitates referrals for specialized genetic and genomic services for patients as needed
• Evaluates impact and effectiveness of genetic and genomic technology, information, interventions, and treatments on patients’ outcome
Human Genome Project and Implications for Clinical Practice
The Human Genome Project was a publicly funded international effort coordinated by the National Institutes of Health (NIH) and the U.S. Department of Energy (www.doegenomes.org). When the Human Genome Project was initiated in 1990, the ultimate goal of the project was to map the human genome (the complete set of genetic instructions in the nucleus of each human cell) by 2005. Considering that the human genome consists of approximately 3 billion base pairs of DNA, many people regarded this as an impossible task. However, by 2003 a substantially complete version of the human genome was announced.
A more recent effort by the National Human Genome Research Institute (NHGRI) called the Encyclopedia of DNA Elements, or ENCODE, was organized to identify the genome’s functional elements. By 2012 researchers “linked more than 80% of the human genome sequence to a specific biological function and mapped more than 4 million regulatory regions where proteins specifically interact with the DNA” (ENCODE, 2012). This made clearer the active genome in which genes are turned on and off by proteins using sites that may be at a great distance from the genes. Identification of regulatory regions will help explain different functions of different types of cells (www.genome.gov/pfv.cfm?pageID=27549810).
Importance of Family History
Completion of the Human Genome Project and the resultant identification of the inherited causes for many diseases have created a renewed interest in family history. Although it is easy to be impressed by the 1900 genetic tests currently available, family history will most likely continue to be the single most cost-effective piece of genetic information. A complete three-generation family history that includes ethnicity information concerning both sides of the family is the best genetic “test” applicable to preconception care. When nurses and other clinicians conduct a family history, they can gain not only valuable information about the structure of the family and diseases that affect various individuals in the family but also a rich understanding of family relationships, social context, occupations, lifestyle, and health habits. The process of collecting this information often facilitates the development of a relationship between the patient/family and the clinician. In 2004 the United States Department of Health and Human Services (USDHHS) launched the Family History Initiative by designating Thanksgiving Day as National Family History Day. The U.S. Surgeon General encouraged families to use their family gatherings as a time to talk about and collect important family health history. A number of family history tools are available free of charge online. One of the most widely used is the My Family Health Portrait (https://familyhistory.hhs.gov). Another family health history tool—Does it run in the family?—was developed by the Genetic Alliance (www.doesitruninthefamily.org).
Gene Identification and Testing
Initial efforts to sequence and analyze the human genome have proven invaluable in the identification of genes involved in disease and in the development of genetic tests. Hundreds of genes involved in diseases such as breast cancer, colorectal cancer, Alzheimer’s disease, and cystic fibrosis (CF) have been identified. The number of commercially available genetic tests continues to increase and can be found on GeneTests, a publicly funded genetics information resource for clinicians (www.ncbi.nlm.nih.gov/sites/GeneTests/).
Most of the genetic tests now being offered in clinical practice are tests for single-gene disorders in patients with clinical symptoms or who have a family history of a genetic disease. Some of these genetic tests are prenatal tests or tests used to identify the genetic status of a pregnancy at risk for a genetic condition. Current prenatal testing options include:
• Maternal serum screening (a blood test used to see if a pregnant woman is at increased risk for carrying a fetus with a neural tube defect or chromosomal abnormalities such as Down syndrome, trisomy 18, and trisomy 13)
• Fetal ultrasound or sonogram (an imaging technique using high-frequency sound waves to produce images of the fetus inside the uterus)
• Invasive procedures (amniocentesis and chorionic villus sampling)
(See Chapter 10 for discussion of these tests.) Other tests are carrier screening tests used to identify individuals who have a gene mutation for a genetic condition but do not show symptoms of the condition because it is an autosomal recessive condition (e.g., CF, sickle cell disease, Tay-Sachs disease).
In addition to using genetic tests for single-gene disorders in patients with clinical symptoms or with a family history of a genetic disease, genetic tests are being used for population-based screening. For example, newborn screening for phenylketonuria (PKU) and other inborn errors of metabolism (IEMs) has been going on in the United States and many other countries for decades (Guttmacher, McGuire, Ponder, et al., 2010). Initially, state-mandated newborn screening in the United States was concerned with only a few conditions. With the advent of tandem mass spectrometry, the number of conditions tested for during newborn screening grew rapidly. Currently, most states use blood spots collected from newborns to test for at least 30 different metabolic and genetic diseases. The conditions most commonly tested for are PKU, congenital hypothyroidism, galactosemia, and sickle cell disease and other hemoglobinopathies. A complete list of conditions tested for in each state is available on the National Newborn Screening and Genetics Resource website (genes-r-us.uthscsa.edu).
Genetic tests are also used to determine paternity, identify victims of war and other tragedies, and profile criminals (www.genetests.org).
Pharmacogenomics
One of most promising clinical applications of the Human Genome Project has been pharmacogenomic testing (the use of genetic information to individualize drug therapy). Associations between genetic variation and drug effect have been observed for a number of commonly used drugs. One of these drugs is warfarin, an anticoagulant commonly used to reduce the risk for thromboembolic events in patients with a history of deep vein thrombosis, pulmonary embolism, myocardial infarction, or atrial fibrillation (Meckley, Gudgeon, Anderson, et al., 2010). There is mounting evidence that genotype-guided warfarin dosing may not only help reduce the serious adverse drug reactions commonly associated with warfarin but also increase dosing accuracy, shorten the time to dose stabilization, and help identify individuals who may require more frequent monitoring.
Gene Therapy
The aim of gene therapy is to correct defective genes that are responsible for disease development. Generally, gene therapy involves inserting a healthy copy of the defective gene into the somatic cells (any cell of the body except sperm and egg cells) of the affected individual. Although the early optimism about gene therapy was probably never fully justified, gene therapy has now moved from preclinical to clinical studies for many diseases. These diseases range from hemophilia and other single-gene disorders to complex disorders such as cancer, HIV, and cardiovascular disorders. Major challenges to gene therapy include determining how to target the right gene to the right location in the right cells, expressing the transferred gene at the right time, and minimizing adverse reactions.
Ethical, Legal, and Social Implications
Because of widespread concern about misuse of the information gained through genetics research, 5% of the Human Genome Project budget was designated for the study of the ethical, legal, and social implications (ELSI) of human genome research. Two large ELSI programs were created to identify, analyze, and address the ELSIs of human genome research at the same time that the basic science issues were being studied. During the past decade, issues of high priority for these programs have been:
• Privacy and fairness in the use and interpretation of genetic information
• Clinical integration of new genetics technologies
• Issues surrounding genetics research, such as possible discrimination and stigmatization
• Education for professionals and the general public about genetics, genetics health care, and ELSI of human genome research
Both ELSI programs have excellent websites that include much educational information, as well as links to other informative sites (www.genome.gov/10001618; www.ornl.gov/sci/techresources/Human_Genome/elsi/elsi.shtml).
Factors Influencing the Decision to Undergo Genetic Testing
The decision to undergo genetic testing is seldom autonomous and based solely on the needs and preferences of the individual being tested. Instead, it is often a decision based on feelings of responsibility and commitment to others. For example, a woman who is receiving treatment for breast cancer may undergo BRCA1/BRCA2 mutation testing not because she wants to find out if she carries a BRCA1 or BRCA2 mutation but, instead, because her two unaffected sisters have asked her to be tested and she feels a sense of responsibility and commitment to them. A female airline pilot with a family history of HD, who has no desire to find out if she has the gene mutation associated with HD, may undergo mutation analysis for HD because she feels she has an obligation to her family, her employer, and the people who fly with her.
Decisions about genetic testing are shaped and, in many instances, constrained by factors such as social norms where care is received and socioeconomic status. Most pregnant women in the United States now have at least one ultrasound examination, many undergo some type of multiple-marker screening, and a growing number undergo other types of prenatal testing. The range of prenatal testing options available to a pregnant woman and her family may vary significantly, based on where the pregnant woman receives prenatal care and her socioeconomic status. Certain types of prenatal testing may not be available in smaller communities and rural settings (e.g., chorionic villus sampling and fluorescent in situ hybridization [FISH] analysis). In addition, certain types of genetic testing may not be offered in conservative medical communities (e.g., preimplantation diagnosis). Some types of genetic testing are expensive and typically not covered by health insurance. Because of this, these tests may be available only to a relatively small number of individuals and families—those who can afford to pay for them.
Cultural and ethnic differences also have a significant impact on decisions about genetic testing. When prenatal diagnosis was first introduced, the principal constituency was a self-selected group of Caucasian, well-informed, middle- to upper-class women. Today the widespread use of genetic testing has introduced prenatal testing to new groups of women, women who had not previously considered genetics services. The fact that many of the women currently undergoing prenatal testing may not share mainstream U.S. views about the role of medicine and prenatal care, the meaning of disability, or how to respond to scientific risks and uncertainties further amplifies the complexity of ethical issues associated with prenatal testing.
Clinical Genetics
Genetic Transmission
Human development is a complicated process that depends on the systematic unraveling of instructions found in the genetic material of the egg and the sperm. Development from conception to birth of a normal, healthy baby occurs without incident in most cases; occasionally, however, some anomaly in the genetic code of the embryo creates a birth defect or disorder.
Genes and Chromosomes
The hereditary material carried in the nucleus of each of the somatic cells determines an individual’s characteristics. This material, called DNA (deoxyribonucleic acid), forms threadlike strands known as chromosomes. Each chromosome is composed of many smaller segments of DNA referred to as genes. Genes or combinations of genes contain coded information that determines an individual’s unique characteristics. The code is found in the specific linear order of the molecules that combine to form the strands of DNA. Genes control both the types of proteins that are made and the rate at which they are produced. Genes never act in isolation; they always interact with other genes and the environment.
All normal human somatic cells contain 46 chromosomes arranged as 23 pairs of homologous (matched) chromosomes; one chromosome of each pair is inherited from each parent. There are 22 pairs of autosomes, which control most traits in the body, and one pair of sex chromosomes. The larger female chromosome is called the X; the smaller male chromosome is the Y. Whereas the Y chromosome is primarily concerned with sex determination, the X chromosome contains genes that are involved in much more than sex determination. Generally, the presence of a Y chromosome causes an embryo to develop as a male; in the absence of a Y chromosome, the individual develops as a female. Thus in a normal female, the homologous pair of sex chromosomes are XX, and in a normal male, the homologous pair are XY.
Homologous chromosomes (except the X and Y chromosomes in males) have the same number and arrangement of genes. In other words, if one chromosome has a gene for hair color, its partner chromosome also will have a gene for hair color and these hair-color genes will have the same loci or be located in the same place on the two chromosomes. Although both genes code for hair color, they may not code for the same hair color. Genes at corresponding loci on homologous chromosomes that code for different forms or variations of the same trait are called alleles. An individual having two copies of the same allele for a given trait is said to be homozygous for that trait. With two different alleles, the person is heterozygous for the trait.
The term genotype typically is used to refer to the genetic makeup of an individual when discussing a specific gene pair, but at times, genotype is used to refer to an individual’s entire genetic makeup or all the genes that the individual can pass on to future generations. Phenotype refers to the observable expression of an individual’s genotype, such as physical features, a biochemical or molecular trait, and even a psychologic trait. A trait or disorder is considered dominant if it is expressed or phenotypically apparent when only one copy of the gene is present. It is considered recessive if it is expressed only when two copies of the alleles associated with the trait are present.
As more is learned about genetics and genomics, the concepts of dominance and recessivity have become more complex, especially in X-linked disorders. For example, traits considered to be recessive may be expressed even when only one copy of a gene located on the X chromosome is present. This occurs frequently in males because males have only one X chromosome; thus they have only one copy of the genes located on the X chromosome. Whichever gene is present on the one X chromosome determines which trait is expressed. Females, conversely, have two X chromosomes, so they have two copies of the genes located on the X chromosome. However, in any female somatic cell, only one X chromosome is functioning (otherwise there would be inequality in gene dosage between males and females). This process, known as X-inactivation or the Lyon hypothesis, is generally a random occurrence. That is, there is a 50-50 chance as to whether the maternal X or the paternal X is inactivated. Occasionally the percentage of cells that have the X with an abnormal or mutant gene is very high. This helps explain why hemophilia, an X-linked recessive disorder, can clinically manifest itself in a female known to be a heterozygous carrier (a female who has only one copy of the gene mutation). It also helps explain why traditional methods of carrier detection are less effective for X-linked recessive disorders; the possible range for enzyme activity values can vary greatly, depending on which X chromosome is inactivated.
Chromosomal Abnormalities
Chromosomal abnormalities are a major cause of reproductive loss, congenital problems, and gynecologic disorders. The incidence of abnormalities is approximately 0.6% in newborns, 6% in stillbirths, and 60% in spontaneous abortions (Martin, 2008). Errors resulting in chromosomal abnormalities can occur in mitosis (cell division occurring in somatic cells that results in two identical daughter cells containing a diploid number of chromosomes) or meiosis (division of a sex cell into two and four haploid cells). These errors can occur in either the autosomes or the sex chromosomes. Even without the presence of obvious structural malformations, small deviations in chromosomes can cause problems in fetal development.
The pictorial analysis of the number, form, and size of an individual’s chromosomes is known as a karyotype. Cells from any nucleated, replicating body tissue (not red blood cells, nerves, or muscles) can be used. The most commonly used tissues are white blood cells and fetal cells in amniotic fluid. The cells are grown in a culture and arrested when they are in metaphase (during metaphase, the chromosomes are condensed and visible with a light microscope), and then the cells are dropped onto a slide. This breaks the cell membranes and spreads the chromosomes, making them easier to visualize. Next, the cells are stained with special stains (e.g., Giemsa stain) that create striping or “banding” patterns. These patterns aid in the analysis because they are consistent from person to person. Once the chromosome spreads are photographed or scanned by a computer, they are cut out and arranged in a specific numeric order according to their length and shape. The chromosomes are numbered from largest to smallest, 1 to 22, and the sex chromosomes are designated by the letter X or Y. Each chromosome is divided into two “arms” designated by p (short arm) and q (long arm). A female karyotype is designated as 46,XX and a male karyotype is designated as 46,XY. Fig. 6-1 illustrates the chromosomes in a body cell and a karyotype.
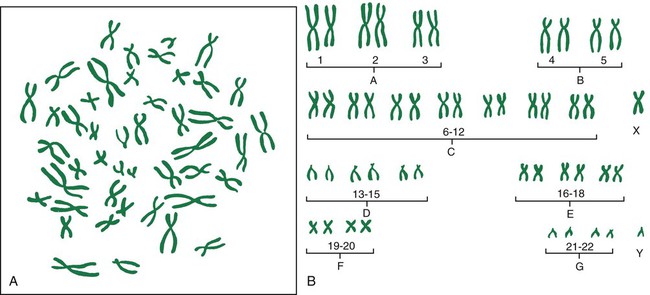
Autosomal Abnormalities
Autosomal abnormalities involve differences in the number or structure of autosome chromosomes (pairs 1 through 22). They result from unequal distribution of the genetic material during gamete (egg and sperm) formation.
Abnormalities of Chromosome Number.
A euploid cell is a cell with the correct or normal number of chromosomes within the cell. Because most gametes are haploid (1N, 23 chromosomes) and most somatic cells are diploid (2N, 46 chromosomes), they are both considered euploid cells. Deviations from the correct number of chromosomes per cell can be one of two types: (1) polyploidy, in which the deviation is an exact multiple of the haploid number of chromosomes or one chromosome set (23 chromosomes); or (2) aneuploidy, in which the numeric deviation is not an exact multiple of the haploid set. A triploid (3N) cell is an example of a polyploidy. It has 69 chromosomes. A tetraploid (4N) cell, also an example of a polyploidy, has 92 chromosomes.
Aneuploidy is the most commonly identified chromosome abnormality in humans and the leading genetic cause of intellectual disability. A monosomy is the product of the union between a normal gamete and a gamete that is missing a chromosome. Monosomic individuals have only 45 chromosomes in each of their cells. The product of the union of a normal gamete with a gamete containing an extra chromosome is a trisomy. The most common autosomal aneuploid conditions involve trisomies. Trisomic individuals have 47 chromosomes in most or all of their cells.
The vast majority of trisomies occur during oogenesis (the process by which a premeiotic female germ cell divides into a mature egg); the incidence of these types of chromosomal errors increases exponentially with advancing maternal age. Although variation exists among trisomies with regard to the parent and stage of origin of the extra chromosome, most trisomies are maternal meiosis I (MI) errors. This means that most trisomies are caused by nondisjunction during the first meiotic division. The first meiotic division involves the segregation of homologous or similar chromosomes. One pair of chromosomes fails to separate. One resulting cell contains both chromosomes, and the other contains none. The fact that most trisomies are maternal MI errors is not that surprising, because maternal MI occurs over a long time span. It is initiated in precursor cells during fetal development, but it is not completed until the time those cells undergo ovulation after menarche.
The most common trisomy abnormality is Down syndrome (DS). Approximately one in every 691 newborns has DS; there are over 400,000 individuals with DS living in the United States (www.ndss.org). Ninety-five percent of individuals with DS have trisomy 21 (nondisjunction) or an extra chromosome 21 (47,XX+21, female with DS; or 47,XY+21, male with DS). Another type of DS, translocation, occurs when extra chromosome 21 material is present in every cell of the individual but it is attached to another chromosome. In the third type of DS, mosaicism, extra chromosome 21 material is found in some but not all of the cells.
Although the risk for having a child with DS increases with maternal age (incidence is approximately 1 in 1200 for a 25-year-old woman; 1 in 350 for a 35-year-old woman; and 1 in 30 for a 45-year-old woman), children with Down syndrome can be born to mothers of any age (www.ndss.org). Eighty percent of children with Down syndrome are born to mothers younger than 35 years. The risk for a mother having a second child with Down syndrome is about 1% when the cause of the Down syndrome is trisomy 21.
Nondisjunction can also occur during mitosis. If this occurs early in development, when cell lines are forming, the individual has a mixture of cells, some with a normal number of chromosomes and others either missing a chromosome or containing an extra chromosome. This condition is known as mosaicism. The most common form of mosaicism in autosomes is mosaic Down syndrome.
Abnormalities of Chromosome Structure.
Structural abnormalities can occur in any chromosome. Types of structural abnormalities include translocation, duplication, deletion, microdeletion, and inversion. Translocation results when there is an exchange of chromosomal material between two chromosomes. Exposure to certain drugs, viruses, and radiation can cause translocations, but often they arise for no apparent reason.
The two major types of translocation are reciprocal and robertsonian. Reciprocal translocations are the most common. In a reciprocal translocation, either the parts of the two chromosomes are exchanged equally (balanced translocation) or a part of a chromosome is transferred to a different chromosome, creating an unbalanced translocation because there is extra chromosomal material—extra of one chromosome but correct amount or deficient amount of the other chromosome. In a balanced translocation, the individual is phenotypically normal because there is no extra chromosome material; it is just rearranged. In an unbalanced translocation, the individual will be both genotypically and phenotypically abnormal.
In a robertsonian translocation, the short arms (p arms) of two different acrocentric chromosomes (chromosomes with very short p arms) break, leaving sticky ends that then cause the two long arms (q arms) to stick together. This forms a new, large chromosome that is made of the two long arms. The individual with a balanced robertsonian translocation has 45 chromosomes. Because the short arm of acrocentric chromosomes contains genes for ribosomal RNA and these genes are represented elsewhere, the individual usually does not show any symptoms. The individual may produce an unbalanced gamete (sperm or egg with too many or two few genes). This can lead to reproductive difficulties such as miscarriages or birth defects.
In duplication, there is an extra chromosomal segment within the same homologous or another nonhomologous chromosome. Clinical findings are highly variable and depend on which of the chromosomal segments are involved.
Deletions result in the loss of chromosomal material and partial monosomy for the chromosome involved. Microdeletions are deletions too small to be detected by standard cytogenetic techniques. Whenever a portion of a chromosome is deleted from one chromosome and added to another, the gamete produced may have either extra copies of genes or too few copies. The clinical effects produced may be mild or severe depending on the amount of genetic material involved. Two of the more common conditions are the deletion of the short arm of chromosome 5 (cri du chat syndrome) and the deletion of the long arm of chromosome 18.
Inversions are deviations in which a portion of the chromosome has been rearranged in reverse order. Few birth defects have been attributed to the presence of inversions, but it is suspected that inversions may be responsible for problems with infertility and miscarriages. More than 40% of inversions involve chromosome 9.
Sex Chromosome Abnormalities
Several sex chromosome abnormalities are caused by nondisjunction during gametogenesis in either parent. The most common deviation in females is Turner syndrome, or monosomy X (45,X). The affected female exhibits juvenile external genitalia with undeveloped ovaries. She is short in stature and often has webbing of the neck, a low hairline in the back, low-set ears, and lymphedema of her hands and feet. Intelligence may be impaired. Most affected embryos miscarry spontaneously. In most cases of Turner syndrome, it is the paternal X or Y that is lost.
The most common deviation in males is Klinefelter syndrome, or trisomy XXY. The affected male has poorly developed secondary sexual characteristics and small testes. He is infertile, usually tall, and effeminate and may be slow to learn (www.genetic.org). Males who have mosaic Klinefelter syndrome may be fertile.
Patterns of Genetic Transmission
Heritable characteristics are those that can be passed on to offspring. The patterns by which genetic material is transmitted to the next generation are affected by the number of genes involved in the expression of the trait. Many phenotypic characteristics result from two or more genes on different chromosomes acting together (referred to as multifactorial inheritance); others are controlled by a single gene (unifactorial inheritance). Specialists in genetics (e.g., geneticists, genetic counselors, and nurses with advanced expertise in genetics) predict the probability of the presence of an abnormal gene from the known occurrence of the trait in the individual’s family and the known patterns by which the trait is inherited.
Multifactorial Inheritance
Most common congenital malformations result from multifactorial inheritance, a combination of genetic and environmental factors. Examples are cleft lip, cleft palate, congenital heart disease, neural tube defects, and pyloric stenosis. Each malformation can range from mild to severe, depending on the number of genes for the defect present or the amount of environmental influence. A neural tube defect may range from spina bifida (a bony defect in the lumbar region of the vertebrae with little or no neurologic impairment) to anencephaly (absence of brain development, which is always fatal). Some malformations occur more often in one sex. For example, pyloric stenosis and cleft lip are more common in males, and cleft palate is more common in females.
Unifactorial Inheritance
If a single gene controls a particular trait or disorder, its pattern of inheritance is referred to as unifactorial mendelian or single-gene inheritance. The number of single-gene disorders far exceeds the number of chromosomal abnormalities. Potential patterns of inheritance for single-gene disorders include autosomal dominant, autosomal recessive, and X-linked dominant and recessive modes of inheritance (Fig. 6-2).
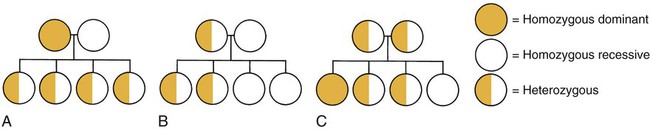
Autosomal Dominant Inheritance.
Autosomal dominant inheritance disorders are those in which only one copy of a variant allele is needed for phenotypic expression. The variant allele may be a result of a mutation—a spontaneous and permanent change in the normal gene structure in which case the disorder occurs for the first time in the family. Usually an affected individual comes from multiple generations having the disorder. An affected parent who is heterozygous for the trait has a 50% chance of passing the variant allele to each offspring (see Fig. 6-2, B and C). There is a vertical pattern of inheritance (i.e., there is no skipping of generations; if an individual has an autosomal dominant disorder such as HD, so must one of his or her parents). Males and females are equally affected.
Autosomal Recessive Inheritance.
Autosomal recessive inheritance disorders are those in which both genes of a pair associated with the disorder must be abnormal for the disorder to be expressed. Heterozygous individuals have only one variant allele and are unaffected clinically because their normal gene overshadows the variant allele. They are known as carriers of the recessive trait. Because these recessive traits are inherited by generations of the same family, an increased incidence of the disorder occurs in consanguineous matings (closely related parents). For the trait to be expressed, two carriers must each contribute a variant allele to the offspring (see Fig. 6-2, C). The chance of the trait occurring in each child is 25%. A clinically normal offspring may be a carrier of the gene. Autosomal recessive disorders have a horizontal pattern of inheritance rather than the vertical pattern seen with autosomal dominant disorders. That is, autosomal recessive disorders are usually observed in one or more siblings but not in earlier generations. Males and females are equally affected. Most inborn errors of metabolism (IEMs), such as phenylketonuria, galactosemia, maple syrup urine disease, Tay-Sachs disease, sickle cell anemia, and cystic fibrosis, are autosomal recessive inherited disorders.
Inborn Errors of Metabolism.
More than 350 inborn errors of metabolism have been recognized (Jorde, Carey, and Bamshad, 2010). Individually, IEMs are relatively rare, but collectively, they are common (1 in 5000 live births). Most IEMs are inherited in an autosomal recessive pattern. IEMs occur when a gene mutation reduces the efficiency of encoded enzymes to a level at which normal metabolism cannot occur. Defective enzyme action interrupts the normal series of chemical reactions from the affected point onward. The result may be an accumulation of a damaging product, such as phenylalanine in PKU, or the absence of a necessary product, such as the lack of melanin in albinism caused by lack of tyrosinase. Diagnostic and carrier testing is available for a growing number of IEMs. In addition, many states in the United States have started screening for specific IEMs as part of their expanded newborn screening programs using tandem mass spectrometry. However, many of the deaths caused by IEMs are the result of enzyme variants not currently screened for in many of the newborn screening programs (Jorde, Carey, and Bamshad, 2010). (See discussion of IEMs in Chapter 25.)
X-Linked Dominant Inheritance.
X-linked dominant inheritance disorders occur in males and heterozygous females, but because of X inactivation, affected females are usually less severely affected than affected males and they are more likely to transmit the variant allele to their offspring. Heterozygous females (females who have one wild-type allele and one variant allele) have a 50% chance of transmitting the variant allele to each offspring. The variant allele is often lethal in affected males since, unlike affected females, they have no normal gene (wild-type allele). Mating of an affected male and an unaffected female is uncommon as a result of the tendency for the variant allele to be lethal in affected males. Relatively few X-linked dominant disorders have been identified. Two examples are vitamin D–resistant rickets and Rett syndrome.
X-Linked Recessive Inheritance.
Abnormal genes for X-linked recessive inheritance disorders are carried on the X chromosome. Females may be heterozygous or homozygous for traits carried on the X chromosome because they have two X chromosomes. Males are hemizygous because they have only one X chromosome, which carries genes with no alleles on the Y chromosome. Therefore X-linked recessive disorders are most commonly manifested in the male with the abnormal gene on his single X chromosome. Hemophilia, color blindness, and Duchenne muscular dystrophy are X-linked recessive disorders.
The male with an X-linked recessive disorder receives the disease-associated allele from his carrier mother on her affected X chromosome. Female carriers (those heterozygous for the trait) have a 50% probability of transmitting the disease-associated allele to each offspring. An affected male can pass the disease-associated allele to his daughters but not to his sons. The daughters will be carriers of the trait if they receive a normal gene on the X chromosome from their mother. They will be affected only if they receive a disease-associated allele on the X chromosome from both their mother and their father.
Genetic Counseling
It is standard practice in obstetrics to determine whether a heritable disorder exists in a couple or in anyone in either of their families. The goal of screening is to detect or define risk for disease in low risk populations and identify those for whom diagnostic testing may be appropriate. A nurse can obtain a genetics history using a questionnaire or checklist such as the one in Fig. 6-3.
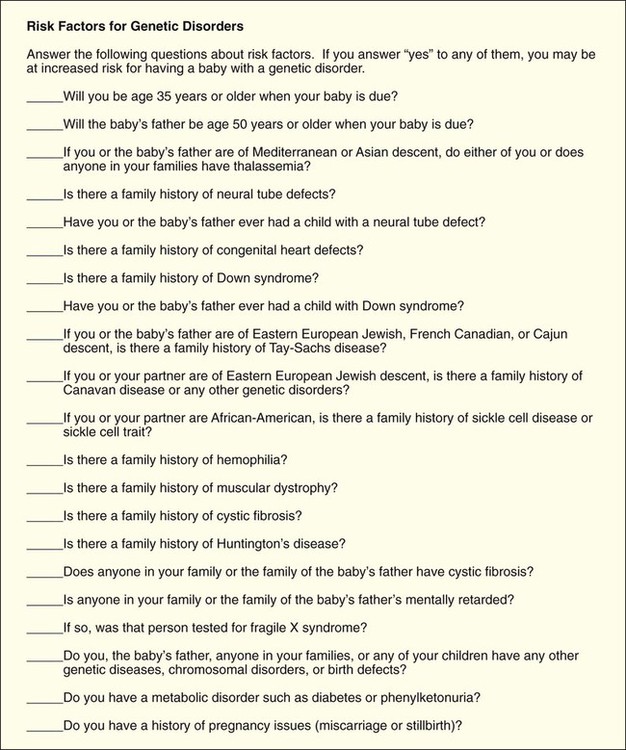
Genetic counseling is a professional service that provides genetics information, education, and support to individuals and families with ongoing or potential genetic health concerns. It is typically provided by a team of genetics specialists that includes clinical geneticists (physicians), medical geneticists with a PhD, genetics fellows, genetics counselors, and, in a growing number of cases, advanced practice genetics nurse specialists. Cytogeneticists, biochemical geneticists, and molecular geneticists support the clinical genetics team by providing laboratory expertise that helps with the diagnosis and management of individuals and families affected by genetic conditions.
Genetic counseling occurs in regional genetics centers, major medical centers, outreach or satellite genetics clinics, public health clinics, some community hospitals, and now that genetics has entered the mainstream of health care, in a wide variety of other settings. These include but are not limited to managed health care organizations, commercial facilities, and private practices. A number of specialized groups provide genetics education and counseling for individuals and families affected by specific genetic disorders, such as DS, CF, diabetes, muscular dystrophy, HD, and cancer. Genetic counseling also is offered over the Internet.
Individuals and families seek out or are referred for genetic counseling for a wide variety of reasons and at all stages of their lives. Some seek preconception or prenatal information; others are referred after the birth of a child with a birth defect or a suspected genetic condition; still others seek information because they have a family history of a genetic condition. Regardless of the setting or the individual’s and family’s stage of life, genetic counseling should be offered and available to all individuals and families who have questions about genetics and their health. However, there is a shortage of appropriately trained genetics professionals who can provide genetic counseling. This means that many individuals and families will not be offered genetic counseling when they undergo genetic testing. Moreover, some of the genetics education and counseling that is provided will be inadequate (see Community Focus box).
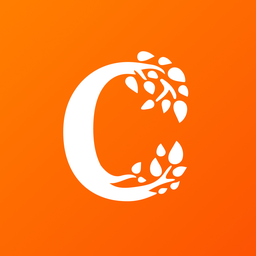
Full access? Get Clinical Tree
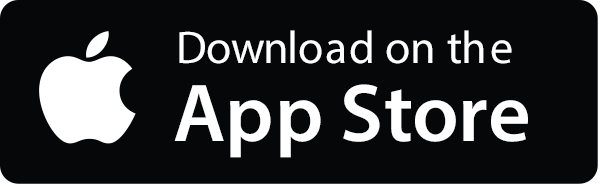
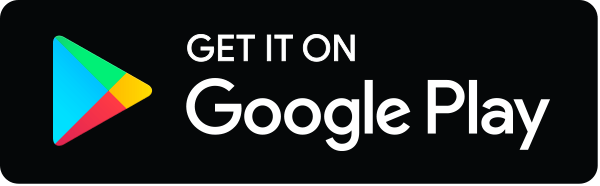