Chapter 45. Caring for children with critical illness
Marion Aylott
LEARNING OUTCOMES
• Demonstrate an understanding of the key concepts of children’s intensive care nursing.
• Explain the principles of respiratory and cardiovascular support within the intensive care setting.
• Consider the role of the children’s nurse as part of the multi-professional team in helping to maintain respiratory support and haemodynamic control.
• Discuss the physical and psychological care of the child with critical illness and their family.
Glossary
Afterload
The load against which the left ventricle ejects after opening the aortic valve.
Cardiac contractility
Contractile capability of the heart.
Cardiac output
The volume of blood that the heart pumps in 1 minute.
Central venous pressure
A measure of pressure in the right atrium.
Chronotropic
Agent that acts to increase heart rate.
Compensated shock
Blood flow is normal or increased and may be maldistributed; vital organ function is maintained.
Compliance
The ease with which the lungs and thorax expand during pressure changes.
Endotracheal intubation
Insertion of endotracheal tube into the trachea either through a patient’s nose or mouth and directly through the larynx between the vocal cords into the trachea to open and maintain airway.
Fibrosis
Replacement of lung epithelium and elastic fibres with fibroplast scar tissue.
Frank-Starling’s law
An increase in preload leads to an increase in stroke volume.
FRC
Functional residual capacity is the pool of air left in the lungs at the end of total expiration.
Heart rate
The number of cardiac contractions that occur in 1 minute.
Hypoxia
An inadequate supply of oxygen.
Inotrope
Agent that acts to improve myocardial contractility and enhance stroke volume.
Irreversible shock
Inadequate perfusion of vital organs; irreparable damage; death cannot be prevented.
Minute volume
Amount of gas inhaled and exhaled in 1 minute.
PEEP/CPAP
Positive end expiratory pressure, or continuous positive airway pressure, is the baseline pressure and controls functional residual capacity in the patient’s lung.
PIP
Peak inspiratory pressure indicates the maximum pressure that has occurred during the last ventilatory cycle.
Preload
The load on the ventricle before ejection.
Shock
Circulatory system failure to supply oxygen and nutrients to meet cellular metabolic demands.
Stroke volume
The amount of blood ejected by both ventricles with each contraction.
Systemic vascular resistance
Tidal volume
The amount of gas or air breathed in or out in one breath.
Uncompensated shock
Microvascular perfusion is compromised; significant reductions in effective circulating volume.
Introduction
A paediatric intensive care unit (PICU) is a specifically designed and equipped multidisciplinary healthcare specialty for the treatment of critically ill children from early infancy to adolescence (British Paediatric Association (BPA) 1993), which has been shown to be important in achieving the best possible outcomes for children (Pearson et al 1997). Nurses are an important element of the team of staff that cares for the critically ill child, and this is reflected by the high nurse:patient ratio – ideally 1:1 – that is characteristic of intensive care. To understand what critical care is, we must focus on the social context in which it occurs. Thus critical care is where care is critical (Coombs 2001). The role encompasses an independent as well as interdependent element. Although critical care nurses work one to one so that they can truly focus on each patient, they will contribute as part of a team when the need dictates. Additionally, nursing and therapeutic intervention in critical care extends beyond the patient. It includes the interface with technology, for example the lines, the monitors, the organ support machinery and the drains.
What is critical illness?
There are around 12,000 admissions each year to PICU in England, which averages approximately 120 in each Health Authority area (NHS Executive (NHSE) 1997a). Critical illness is a derangement in physiology with the potential to result in significant morbidity and mortality without prompt and appropriate invasive and therapeutic intervention (Hazinski 1999). There are, however, many differences in healthcare facilities available in PICUs across the UK relating to the case mix of patients admitted and the intensive care resources available in particular units. The variety of disease seen in any children’s intensive care unit depends on the population from which it receives patients (Duncan 1998). For example, a hospital with a cardiac surgery programme will increase the number of patients that unit admits with that condition. Although a wide variety of diseases may lead to critical illness, the fundamental interventions required are limited. The common pathway of deterioration in critical illness occurs as a result of progressive deterioration of respiratory and circulatory function with respiratory failure or shock as the pathophysiological expression with the final denominator being cardiopulmonary failure (Advanced Life Support Group (ALSG) 2001).
What constitutes an intensive care patient?
As we have seen, critical illness is characterised by acute loss of physiologic reserve but in many cases the course of illness is prolonged and often the underlying cause may be difficult to discern. Moreover, despite a child having a recognised critical illness there is great interpatient variability. In addition, until the very recent past children with critical illness were nursed in a range of settings including general adult intensive care units depending on geographical location. In Britain, paediatric intensive care developed in an ad hoc and fragmented way. Now, however, after 20 years of effort, Britain is moving towards a more integrated service.
Concerns about paediatric intensive care were first raised by the British Paediatric Association (BPA) in the 1980s, and again in 1993 when it published its report on paediatric intensive care. This report highlighted the fragmented configuration of paediatric intensive care provision, demonstrating that only 51% of children were cared for in paediatric intensive care units, 20% in adult intensive care units and 29% in children’s wards (BPA 1993). Furthermore, only 36% of paediatric intensive care units provided a retrieval service. Concerns were also expressed regarding the lack of physical facilities, lack of education and training of both medical and nursing staff and poor staffing levels available to care for these critically ill children.
In 1996 an inquiry into the death of a child named Nicholas Geldard, who died in a paediatric intensive care unit in 1995 after inappropriate transport for management following a spontaneous cerebral haemorrhage, was published (Ashworth 1996). In response to this, the Department of Health (DoH) requested an inquiry into paediatric intensive care services with a view to developing a policy framework for paediatric intensive care (NHSE1996). In 1997 ‘Paediatric intensive care: a framework for the future’ was published (NHSE 1997a). This paper set out a strategy for developing and integrating the service for critically ill children within a geographical area and so centralise the skills and experience of medical and nursing staff. The central recommendations of this paper have been supported by others (Pearson et al 1997).
Today, children requiring intensive care are no longer cared for in general children’s wards and centres that do not meet the standards as laid out by the NHSE framework document. Quality of paediatric intensive care is not only reliant on a centralised and standardised service but also – importantly – the effectiveness and appropriateness of treatment within a child- and family-orientated environment. To facilitate this, nurses working in paediatric intensive care units undertake a specifically designed, competency-based course to equip them with the knowledge, skills and understanding that underpins effective paediatric intensive care nursing practice.
www

Read the DoH papers Framework for the future and A bridge to the future online:
Children are admitted to PICU when one or more body systems cannot maintain physiological homeostatic equilibrium without intensive therapeutic support. However, there are varying degrees of organ failure and levels of intensive support (NHS Executive, 1997a and NHS Executive, 1997b).
Activity
Scenario
Activity
Scenario

Analyse the categories of intensive care as defined by the DoH papers: A framework for the future and A bridge to the future. Discuss in your learning group how these levels of care and facilities, including staff skills and expertise, compare with a general paediatric ward.

Introducing Daniel
The Badger ward paediatrician and nursing staff were concerned about the condition of 15-month-old Daniel, who weighed 13 kg and was developing increased respiratory distress since admission 4 hours earlier. Daniel had been admitted to Badger ward as a referral from his GP and was accompanied by his parents, Emma and Alan. Daniel had a 2-week history of an upper respiratory tract infection, which over the previous 2–3 days had worsened, with decreased appetite and fluid intake. Since admission to Badger ward, his respiratory distress had markedly increased. Initial treatment was directed towards alleviating his respiratory distress and included 30% oxygen via a head box and intravenous antibiotics. Despite this therapy there had been no evidence of improvement. The paediatrician contacted the PICU intensivist to review Daniel on Badger ward.
The paediatric intensivist and an experienced PICU nurse equipped with emergency bag and retrieval trolley responded immediately to this request. On assessment they found that Daniel was in obvious respiratory distress. He was irritable and reluctant to leave his mother’s arms. His pulse rate was 180, respiratory rate 65 with grunting and sternal and intercostal chest recession. Inspiratory stridor and expiratory wheeze were readily audible and his tidal volume was decreased.
Daniel was transferred to PICU on the retrieval trolley on his mother’s lap.

Consider Daniel’s situation and the DoH categories for intensive care. What was the rationale for Daniel’s admission to PICU?
Relating back to Chapter 27, consider why the critical care team who assessed Daniel decided to transfer him to PICU in his mother’s lap, without further intervention on Badger ward.

On admission to PICU, additional vital signs were obtained:
• Daniel was awake and irritable and appeared exhausted
• respiratory rate had increased from 60 to 70
• his recession appeared pronounced
• oxygen saturation: 95%
• pulse rate had increased from 174 to 200
• radial and dorsalis pulses were weak and thready
• his skin was cool, dry and pale
• capillary refill: 4 seconds
• blood pressure: 90/60 mmHg
• he was conscious and responding to his mother
• blood glucose: 1.7 mmol/L
• axilla temperature: 38.5°C
• no skin rash noted
• an arterial gas was obtained: pH 7.25, pCO 2 7.6, pO 2 22, base deficit −3.
Rapid cardiopulmonary assessment
Recognition of potential respiratory failure, based on clinical evaluation of the child is vital (ALSG 2001). Intubation and ventilation of a child with potential respiratory failure will be considered if a child fails to improve after initial oxygen therapy or if further deterioration is observed. An individual must be able to support three specific functions:
• Protect the airway
• Adequately ventilate
• Adequately oxygenate.
A failure to perform any one function will result in respiratory failure. Respiratory failure is defined as a major abnormality of gas exchange (Lissuer & Clayden 2001) and is an absolute indication for intubation and mechanical ventilation. However, not every patient who is intubated has a primary pulmonary pathology. Intubation and ventilation may serve to support the cardiovascular system. For example, for patients in cardiogenic shock the demands of the respiratory system may precipitate cardiovascular collapse. Supporting the patient with mechanical ventilation can reduce the demands on the heart, allowing it to recover. Intubation can also serve to protect the airway for those who cannot do it themselves, for example patients in coma as a result of a head injury. Moreover mechanical ventilation offers the option of hyperventilation for patients with intracranial hypertension.
Activity

Analyse the data collected during a rapid cardiopulmonary assessment of Daniel and arterial blood gas and list the factors that support the decision to intubate and ventilate.
Respiratory support
Intubation
Intubation and ventilation are always performed with caution, as anaesthetic agents as well as positive pressure ventilation will exacerbate hypovolaemia. Assessment of Daniel’s perfusion indicates a degree of hypovolaemia therefore he was given 260 ml (20 mL/kg) of normal saline as an intravenous bolus with good effect observed by an improvement in his heart rate and perfusion. In addition, he was administered broad-spectrum intravenous antibiotics.
Intubation is a painful and unpleasant procedure and should never be performed on a conscious or semi-conscious patient. Unless the child has completely collapsed, the medical practitioner, usually an anaesthetist or intensivist, will perform what is called rapid sequence induction. This means inducing anaesthesia and giving muscle relaxants. Common drugs used in this age group are morphine, midazolam and atracurium. When the drugs are being prepared for rapid sequence induction, equipment should be prepared and checked to be in good working order to ensure a safe procedure, as displayed in Figure 45.1.
![]() |
Fig. 45.1 The action and rationale for preparation prior to intubation. |
Mechanical ventilation: physiological principles and effects
Mechanical ventilatory support is the major supportive treatment used in critical care. A mechanical ventilator is simply a machine used to replace or supplement the natural function of breathing. It is usually a temporary measure until the patient can breathe adequately without help. Ventilation is simply the movement of gas into and out of the lungs. To safely and effectively provide care for intubated infants and children receiving assisted ventilation it is necessary to have an understanding of the fundamental physiological principles of lung function and the physiological effects of assisted ventilation in terms of both gas exchange and pulmonary mechanics and an appreciation of the particular disease process leading to the need for assisted ventilation.
Ventilation and perfusion relationship
The primary function of the lungs is to enable gas exchange between inspired air that reaches the alveoli and the blood of the pulmonary capillaries. This process of gas exchange is crucial for homeostasis and is interdependent with the circulatory system’s prime role of blood transport. To enable adequate gas exchange it is crucial that oxygen and carbon dioxide move between air and blood by simple diffusion across the alveolar surface. More specifically, there is a balance between ventilation (V) and perfusion (Q). However, it is more complicated than this. V and Q are not evenly distributed throughout the lung (Hazinski 1999). Figure 45.2 illustrates how V increases from apex to the base of the lung and Q increases from apex to base relatively more than perfusion. During normal quiet breathing in an upright position the bases of the lung receive about 50% more ventilation than the apices. This is due to:
• gravity
• variations in airways size (remember Poiseuille’s law, Chapter 4)
• variations in alveolar elastic properties (surfactant – to be discussed later).
![]() |
Fig. 45.2 The ventilation (V) and perfusion (Q) relationship. |
If oxygen is not delivered to alveolar capillaries, or the alveolar surface is damaged, oxygen and/or carbon dioxide elimination may be impaired, V and Q are not balanced and respiratory failure ensues. In a single lung unit V and Q must be matched to allow gas exchange (Fig. 45.3).
![]() |
Fig. 45.3 A simple illustration of V and Q mismatch. |
Mechanical ventilation is an attempt to normalise the patient’s ventilation-perfusion ratio. However, perfusion is determined by:
• the amount of oxygen in the blood
• the oxygen binding capacity of haemoglobin
• cardiac output.
Therefore, when a patient is being ventilated, therapeutic interventions must also be taken to optimise perfusion through ensuring there is a sufficient delivery of inspired oxygen, concentration of haemoglobin and cardiac output. We will look at cardiac output in more detail later.
The mechanics of breathing
Three factors affect the mechanics of breathing:
• Force (pressure)
• Displacement (volume)
• Rate of displacement (flow), which comprises compliance and resistance.
These factors are all interrelated:




During normal inspiration the mechanical contraction of the inspiratory muscles causes the thoracic cage to enlarge in volume. This increased volume causes a pressure gradient between the lungs and the atmosphere. This pressure differential is enough to cause an in-rush of air into the lungs. The work that the inspiratory muscles perform represents the negative pressure applied. Figure 45.4a shows the changes in airway pressure in the mouth during spontaneous quiet breathing. Airway pressure during inspiration is negative, allowing air to enter the lungs, and is positive during expiration. Also, there is normally a brief pause, when airway pressure remains at atmospheric pressure. Figure 45.4b shows the upper airway pressures (measured at the mouth) during mechanical ventilation. In artificial ventilation, the ventilator must provide the inspiratory pressure provided by the inspiratory muscles in normal respiration. This is done by creating a positive intrapulmonary pressure whereby air is pushed out of the ventilator into the lungs of the patient, thus raising the pressure in the airways relative to atmospheric pressure. This resultant increase in intrapulmonary pressure forces the lungs to expand. It is important to remember that artificial ventilation fundamentally alters normal airway pressures. This fact accounts for most of its benefits and complications.
![]() |
Fig. 45.4 Changes in airway pressure during (a) normal spontaneous breathing versus (b) artificial mechanical ventilation. |
In the equation Pressure = Volume ÷ (Compliance + Resistance), compliance and resistance are assumed to remain constant and is illustrated by (a) in Figure 45.5. However, this is not the case in cardiopulmonary illness. With stiff lungs (reduced compliance) patients tend to take rapid, small breaths, to minimise elastic workload as in Figure 45.5b. With high airway resistance, for example in asthma, patients take large slow breaths (Figure 45.5c). As can be seen in Fig. 45.5, the inspiratory lung volume is low in both. Thus, their combined effect is a greater load presented to the ventilator. Pressure, volume and flow change over time in illness, therefore they are considered variable. From the equation of motion, the ventilator must control one of these variables. A mechanical ventilator will control airway pressure, inspired volume or inspired flow.
![]() |
Fig. 45.5 The effects of compliance and airway resistance on lung volume. (a) The effect of normal compliance; (b) the effect of reduced compliance; (c) the effect of increased airways resistance. |
But there is more to compliance. To achieve compliance, alveolar lung units have an important lung property – they are elastic. They can be expanded by small forces and return to their resting state. Two components are responsible for their elastic behaviour:
Thus less pressure is required to inflate alveoli (Martini 1998). The lung contains a foamy phospholipid fluid commonly known as surfactant. Pulmonary surfactant is produced by type I and II alveolar pneumocytes, and is responsible for decreasing the surface tension of the lining fluid within the bronchial tree. Without surfactant, pulmonary airways become unstable and have decreased compliance. Surfactant decreases the surface tension of the alveoli in order to aid the diffusion of gases (Martini 1998). A reduction in production of surfactant will directly affect gas exchange. General causes of reduced surfactant production are:
• acidosis
• hypoxia
• hyperoxygenation
• pulmonary oedema
• atelectasis.
To help understand this concept, think about blowing up a balloon. At first it is difficult and then there is a ‘give’ and the balloon inflates easily. This occurs in the same way in the lungs. The greatest workload in the respiratory cycle is in early inspiration where the pressure–volume curve is relatively flat (Fig. 45.6).
![]() |
Fig. 45.6 Alveolar compliance and the pressure–volume curve. |
Once the steep part of the pressure–volume curve (PVC) (the P flex) is reached, the lung suddenly inflates easily; this is the inflection point. The PVC is flattest at low lung volumes and consequently the work of breathing is highest. If the lungs were left filled with air so that the resting state is at or above the inflection point, the work of breathing is reduced considerably. Going back to our analogy, this is like letting a balloon deflate incompletely and then re-inflating it. This is the basis for positive end expiratory pressure (PEEP), which is a continuous constant pressure of gas delivered to prevent alveolar collapse (PEEP will be discussed in more detail later).
Finally, the last physiological mechanism we must consider is closing volume. In health, the lungs dangle downwards in the pleural cavity supported by a vacuum of negative pressure. As you would expect, the magnitude of this negative pressure is greater in the apices than in the bases. The effect of this is to splint the airways open at the end of the tidal volume (normal expiration) – the expiratory reserve volume (ERV) (McCance & Huether 2002) (Fig. 45.7). Apical alveoli are more inflated at rest than basal. But during inspiration, because the latter are at a steep part of the pressure–volume curve, the gas turnover (see Fig. 45.6) is greater. However, in illness, further confounded by upper airway compromise, and especially when lying supine, there is a tendency for distal, particularly basal airways to collapse in expiration. This is known as the closing volume. If this is not addressed by delivering PEEP through the ventilator, eventually the closing volume will impinge on and begin to exceed functional residual capacity (FRC) and atelectasis (alveolar collapse) will occur at the end of quiet expiration.
![]() |
Fig. 45.7 Lung volumes. |
As we have seen, artificial ventilation is unlike normal ventilation. Positive pressure ventilation can be injurious to the lungs, which were designed as a negative pressure circuit receiving oxygen at 21%. Moreover, artificial ventilation is most often required by patients with underlying lung or cardiovascular pathology which makes the lung tissue even more susceptible to ventilator-induced lung injury and cardiovascular complications.
Evidence-based practice

Evidence has been mounting since the late 1980s that mechanical ventilation can cause and exacerbate lung tissue injury (Dreyfus et al., 1985 and Kolobow et al., 1987). Although most of this evidence has originated from animal studies, clinical reports support this concern (Bohn 1998):
• Oxygen toxicity
• Barotrauma (pressure)
• Volutrauma (shearing forces)
• Insufficient humidification.
Ventilation: lessons learned
Accumulating evidence has revealed that high oxygen concentrations, high inflation pressures, large tidal volumes and low PEEP ventilation strategies are damaging to the lungs:
• Oxygen in supraphysiological concentrations is injurious to the lung, particularly in concentrations over an FiO 2 of 0.60 (Robb 1997a).
• Positive pressure ventilation is non-physiological and causes pulmonary injury. The higher the pressures used, the greater the injury. This is referred to as barotrauma and is typically associated with excessive airway pressures leading to extra-alveolar air and also includes pneumothorax, pneumomediastinum, pneumoperitoneum and subcutaneous emphysema. What exactly qualifies as a dangerous pressure is not known (Bohn 1998).
• Volutrauma is lung injury secondary to alveolar overdistension. It is manifested by disruption of the alveolar capillary membrane and alterations in gas exchange. The propensity for volutrauma is related to the volume of lung available and to regional differences in compliance. In, for example, pneumonia where lung volume is significantly diminished and compliance is reduced, the risk of volutrauma is increased (Kerr 1997).
• Shearing forces caused by repeated expansion of collapsed alveoli is injurious to the lung by causing the release of inflammatory mediators independent of over-distension or oxygen concentration (Bohn 1998). The concept of ‘shear’ injury along with the increase in dead space to tidal volume ratio prevents the use of high rates to compensate for small tidal volumes/low pressures.
Strategies to reduce lung injury
In response to these findings, respiratory experts have espoused the use of lung protective ventilator strategies (Kerr 1997). One strategy is to use sufficient PEEP to raise FRC above closing capacity without compromising cardiac output and as a consequence oxygen delivery. This strategy keeps alveolar units on the steep part of the compliance curve (see Fig. 45.6). Higher levels of PEEP help to maintain:
• alveolar recruitment (the ‘open lung’ approach)
• reduce V/Q mismatch and progressive hypoxemia
• achieve lower tidal volumes (4–8 mL/kg).
Thus, higher levels of PEEP lead to reduced end inspiratory volumes preventing shear/stretch injury.
Another strategy advocated is the use of pressure-limited ventilation and increasing mean airway pressures by permissive hypercapnia and hypoxemia (Reynolds et al 1993). There is no research evidence to dictate what acceptable oxygen or carbon dioxide levels are. The general philosophy is that an acceptable level is determined by what intensivists are willing to do to get there considering the research findings available regarding the causation of lung injury. For example, if an oxygen saturation of 95% can only be achieved through using a PEEP of 12 cmH 2O and a FiO 2 of 1.0 (100%) this is not acceptable in the light of the best available evidence. Both high PEEP and high inspired-oxygen concentrations are injurious to the lung. However, if achieving a carbon dioxide level of 5.4 kPa results in a peak pressure of 30 cmH 2O, this might be acceptable as this carbon dioxide level is acceptable. In general (Parker et al 1993):
• peak pressures greater than 35 cmH 2O are not acceptable
• the upper limit for an acceptable FiO 2 is 0.60
• the upper PEEP limit is 15 cmH 2O.
It is recognised that these limits are arbitrary in nature and no study has ever demonstrated what safe limits are (Hill 1997). These limits are made by intensivists in the context of the patient’s clinical situation and expected clinical course. Thus the overall goal of mechanical ventilation is not to achieve a target blood gas value but to maximise oxygen delivery while minimising pulmonary injury.
Inhaled nitric oxide (iNO) was originally thought to be the ‘magic bullet’ for improving V/Q mismatch in severe respiratory failure (Tibballs 1998). Given its ability to vasodilate blood vessels, be delivered as a gas (act on blood vessels that perfuse ventilated alveoli: selective pulmonary vasodilatation) and its short half-life (no systemic hypotension), it was studied with great interest. However, although iNO does improve oxygenation in acute respiratory distress syndrome, it does not improve outcome. It has more of a beneficial effect in patients with pulmonary hypertension, especially neonates with persistent pulmonary hypertension of the newborn or post-operative cardiac patients.
There are options, beyond adjusting the ventilator, which have implications for nursing. Body positioning affects ventilation/perfusion (V/Q) matching and therefore arterial oxygen levels. Accumulation of secretions and atelectasis can occur in the dependent regions of the lungs, therefore regular position changes will help to avoid problem areas developing. Position can also affect a child’s FRC. A reduction in FRC will make a child more vulnerable to atelectasis and allow more rapid hypoxia. It is important to consider which positions may help to optimise oxygenation and this is a multi-professional decision made in consultation with a respiratory physiotherapist. It is well established that atelectasis develops in the dorsal areas of the lung when patients are in a supine position for any extended period of time (Ganong 2001). ‘Prone positioning’ a patient can help re-expand these collapsed areas, improve alveolar ventilation and hence gas exchange (Ball et al 2001). Additionally, the chest wall has a more favourable compliance curve in the prone position. Most patients will usually have improved oxygenation when prone and can tolerate being prone for 20 hours at a time, with regular pressure relief. It has not been established that prone positioning improves mortality but it can be useful in the patient that is difficult to oxygenate.
In normal circumstances the upper airway warms and humidifies air, so that it reaches core temperature and 100% saturation just below the carina. This process is known as gas conditioning (Williams et al 1996). During gas conditioning inspired air is also cleaned and filtered of foreign particles by the mucociliary transport system, which extends from the nasopharynx towards the bronchioles (Estes & Meduri 1995). Thus, gas conditioning optimises gas exchange and protects delicate lung tissue. The placement of an endotracheal tube bypasses this defence mechanism and the gas exchange function of the lung. The placement of the endotracheal tube physically shifts the conditioning, and heat and moisture recovery functions, further down the airway which would not normally be required to give up heat and moisture.
Hence in the intubated patient, the mucociliary transport system is the sole remaining mechanical defence system. The mucociliary transport system’s function depends on the:
• thickness of the mucus
• depth of the aqueous layer
• cilia beat frequency.
If the temperature of inspired gas is less than core temperature the beat frequency of the cilia will reduce and the gas will be heated to a suboptimal temperature causing the relative humidity of the gas to be reduced. Less than optimal humidification results in a compromised mucociliary transport and pooling of mucus in the lower airways thus restricting gas exchange and being an ideal site for bacterial colonisation (Centers for Disease Control (CDC) 1994). Furthermore, the gel layer will lose moisture and become thicker. If suboptimal humidification is allowed to continue, cell damage might occur and the process of gas conditioning will move deeper into the lung. Increased thick mucus and reduced clearance of secretions from small airways results in reduced airway patency and lung compliance (Williams et al 1996). Thus the nurse must ensure that inspired gases are maintained at core temperature and humidity. Effective humidification will also maximise lung defence by reducing exposure to contaminants invading the airways by increasing clearance of contaminants through suctioning.
Cardiovascular complications: lessons learned
Mechanical ventilation through its delivery of positive intrathoracic pressure has an adverse effect on the heart. Consequently, patients require close assessment and monitoring of their cardiovascular function. Increasing intra-thoracic pressure decreases venous return to the right side of the heart and subsequently to the left side of the heart (Robb 1997b). Therefore patients may need additional fluid volume to maintain cardiac output. The nurse must be mindful to observe cardiovascular parameters in relation to ventilator airway pressure. Decreased cardiac output due to positive pressure ventilation is often ameliorated by fluid administration. The degree of cardiac compromise may often limit the amount of PEEP that a child can tolerate and hence what the intensivist is willing to use.
Modes of ventilation
The goals of assisted ventilation are to achieve adequate gas exchange while at the same time minimising damage to the lungs or interference to the circulation. The review of the risks and side effects of positive pressure ventilation possibly goes some way towards explaining why there are quite so many modes of ventilation available today.
This chapter will now turn to a brief synopsis of the common approaches to ventilation based on physiological principles. It is by no means exhaustive or complete, and is not intended to be. For a more exhaustive discussion of management issues surrounding mechanical ventilation of the sick child refer to one of the major intensive care texts, such as the ‘Manual of pediatric critical care’ (Hazinski 1999).
Ventilators (Fig. 45.8) deliver gas to the lungs using positive pressure at a certain rate. The amount of gas delivered can be limited by time, pressure or volume. The duration can be cycled by time, pressure or flow. The basic terms used with ventilators are the following:
• Peak inspiratory pressure (PIP): indicates the maximum pressure that has occurred during the last ventilatory cycle
• Positive end expiratory pressure (PEEP): is the baseline pressure and controls functional residual capacity (FRC)
• Pressure above PEEP (PAP)
• Mean airway pressure (MAP)
• Inspiratory time (Ti): shown in seconds
• Tidal volume (Vt): amount of gas delivered with each breath
• Minute volume (MV): amount of gas breathed in or out over 1 minute = Vt ∞ Rate
• Continuous positive airway pressure (CPAP).
![]() |
Fig. 45.8 A ventilator (top) and a close-up of the control panel (below). |
Note: CPAP is equivalent to PEEP, except the term is usually used when referring to patients who are not intubated but on what is called nasal CPAP (NCPAP).
The common ventilation modes illustrated in Figure 45.9 are the following:
• Assisted control mode (AC): the ventilator will guarantee that the patient receives a set tidal volume or peak airway pressure with every breath. The patient can breathe ‘above’ the set rate but will receive full support regardless of their effort.
• Intermittent mandatory ventilation (IMV): the ventilator supports breaths only at the set rate and interval. Breaths ‘above’ the set rate are not supported.
• Synchronised intermittent mandatory ventilation (SIMV): the ventilator synchronises an IMV ‘breath’ with the patient’s effort. If, for example, the set rate is 10, then every 6 seconds the patient will receive a machine triggered breath. In between those 10 breaths, the patient is free to breathe but those breaths are not supported. These breaths can be supported if a ‘pressure support mode’ is added.
• Pressure support or pressure control mode (PS/PC): the ventilator supplies pressure support but no set rate. A patient needs to generate a certain amount of work to trigger it. Additionally, a patient has to breathe through an endotracheal tube that is almost always narrower than their own airway and ventilate the increased dead space imposed by the ventilator circuit. A patient may not be able to generate adequate tidal volumes for these reasons. To compensate for this increase in the work of breathing, pressure support is given.
![]() |
Fig. 45.9 Ventilator modes. |
Irrespective of mode, whenever a breath is supported by the ventilator, the limit of the support is determined by a preset pressure or volume. Thus, if volume is set, pressure varies and conversely, if pressure is set, volume varies according to the patient’s lung compliance.
In CPAP, PEEP is elevated baseline airway pressure (Fig. 45.10). The two terms ‘PEEP’ and ‘CPAP’ are used interchangeably and can lead to confusion. They are in fact the same thing. The concept of PEEP is that a pressure is applied at the end of expiration to maintain alveolar recruitment and can, therefore, improve oxygenation and ventilation. Airway pressure is kept positive and not allowed to return to atmospheric. PEEP is usually set at between 4 and 10 cmH 2O, which is usually enough to ensure that the patient receives sufficient tidal volume (Pearson 2002). It can also stent open areas of trachea malacia (abnormal softening) and thereby improve ventilation and oxygenation even if these areas in of themselves do not participate in gas exchange. A great advantage is that CPAP can be delivered non-invasively either by nasal prong or mask (Fig. 45.11).
![]() |
Fig. 45.10 CPAP – pressure wave. |
![]() |
Fig. 45.11 CPAP driver. |
As ventilator technology has advanced, newer modes have been developed such as, pressure-regulated volume control (PRVC), volume support, inverse ratio (IRV), airway-pressure release ventilation (APRV) and high-frequency oscillatory ventilation (HFOV). Some are variations of volume or pressure modes and some are completely unrelated to conventional mechanical ventilation. It is important to recognise that none of these modes has yet been shown to be better than another or to reduce mortality for any disease.
Ventilator settings
The initial settings prescribed and set by the intensivist for both volume and pressure are similar. Except in volume control a tidal volume is set at usually 8–10 mL/kg and in pressure control the peak airway pressure is set. The starting peak airways pressure is set at what is needed to adequately inflate the patient’s chest and generate breath sounds and is usually 15–20 cmH 2O above PEEP. The starting breath rate is usually one that would be physiologically appropriate for the age of the patient. Immediately after intubation patients are usually placed on a FiO 2 of 1.0 and are weaned down as long as the oxygen saturations remain acceptable. PEEP is usually set at 5 cmH 2O and then increased as needed to achieve acceptable oxygen saturations with a FiO 2 less than 0.6 (Pearson 2002) (Fig. 45.12).
![]() |
Fig. 45.12 Simple paradigm to explain likely ventilation setting changes. |
Respiratory assessment and monitoring of the ventilated child
Clinical observation of the ventilated child is mandatory. Generally it is the intensive care nurse who observes, assesses, communicates, monitors, analyses and interprets data in order to effectively care for the critically ill child 24 hours a day (Fig. 45.13). In addition to the usual respiratory and cardiovascular observations discussed in Chapter 4, the critical care team must observe adaptation of the ventilator to the child and the degree of harmony between these two partners.
Activity
Activity

To ensure patency of the airways, the nurse must ensure that active humidification is set to administer inspired gas at 37°C and 100% relative humidity in the lungs to assist lung clearance of secretions from the endotracheal tube by suctioning using an evidence-based technique. Trauma, infection and cardiopulmonary compromise are well-documented adverse events associated with a poor technique:
• Read the review by Pollard (2001).

Figure 45.12 presents a simple paradigm to help explain how medical practitioners decide which ventilation parameter to alter based on the results of an arterial blood gas. Use the figure to determine what parameters might be altered by the intensivist in response to the arterial blood gas below taken 4 hours after Daniel was intubated and ventilated:
• pH 7.56, pCO 2 3.2, pO 2 20.0, base deficit −5
• oxygen saturations were 100%.
Analyse your local intensive care observation chart and note the breadth of data that are collected at least hourly.
Consider the similarities and differences to the data you would collect on a child receiving oxygen therapy on a children’s medical ward.
![]() |
Fig. 45.13 Nursing assessment. |
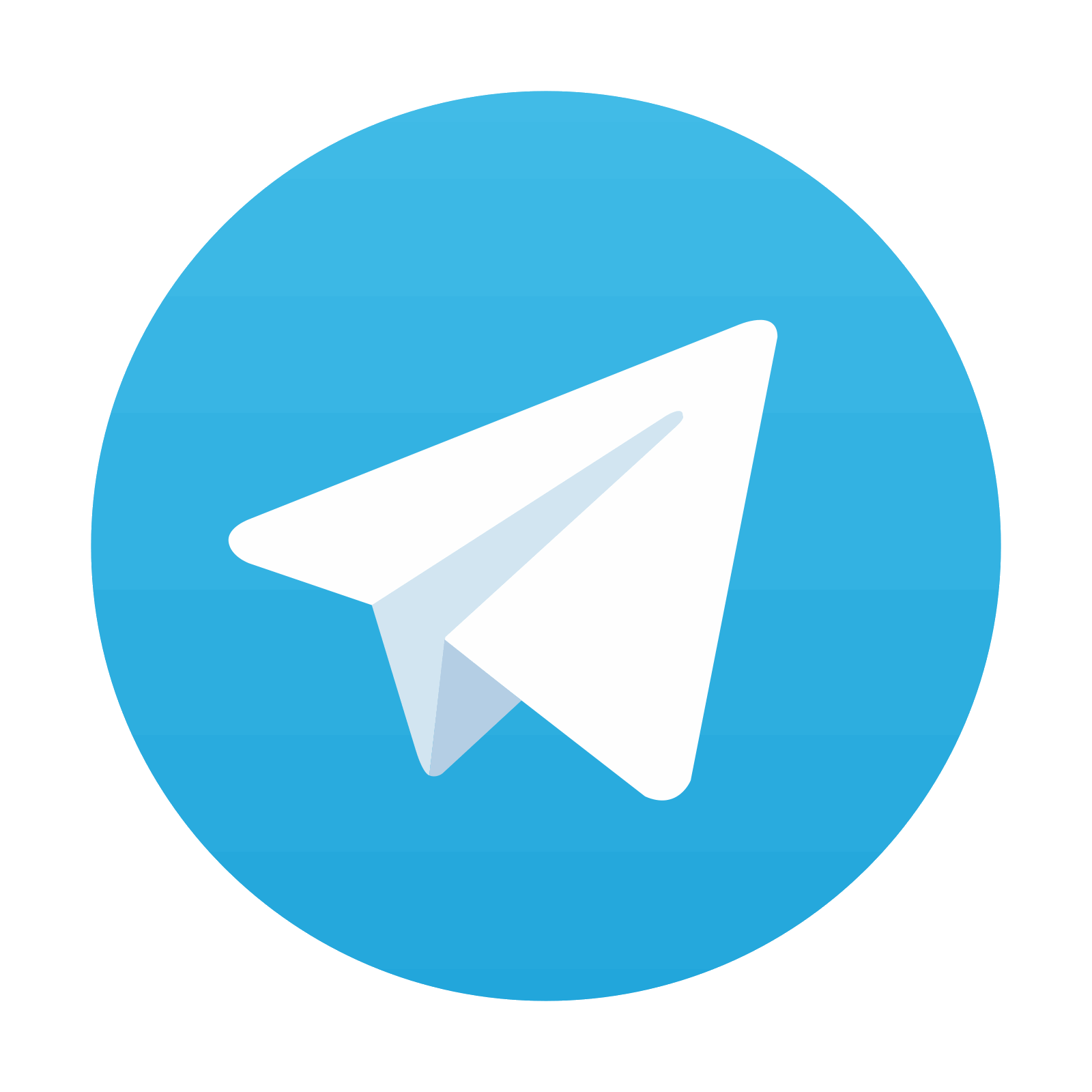
Stay updated, free articles. Join our Telegram channel
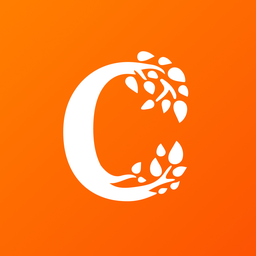
Full access? Get Clinical Tree
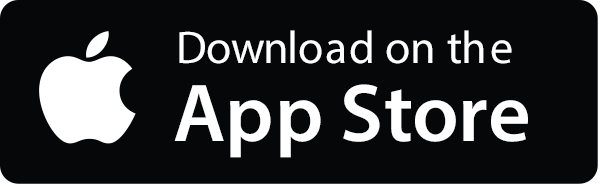
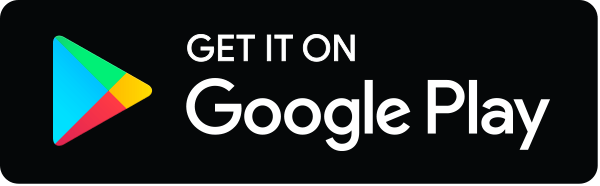
Get Clinical Tree app for offline access
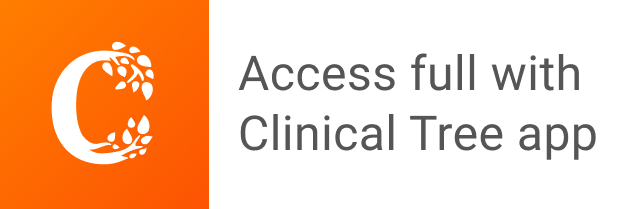