CHAPTER 8 1. Identify the influences on fluid and electrolyte homeostasis in the newborn infant. 2. Describe fluid and electrolyte management in the neonate. 3. Compare fluid and electrolyte management of the full-term infant and the prematurely born newborn. 4. Discuss acid–base balance in the neonatal period. An essential part of the successful transition to extrauterine life is the achievement of fluid, electrolyte, and acid–base homeostasis and control. Because mature control of these processes may not occur for days to weeks after birth, premature and other stressed neonates can have transient disturbances of fluid, electrolyte, and acid–base balance. A. Fluid homeostasis in the fetus and neonate. 1. Body water distribution. Water, the most abundant component of the body, is distributed in two main compartments: intracellular fluid (ICF) and extracellular fluid (ECF); the latter is composed of intravascular and interstitial spaces. As gestation progresses, the fetus undergoes changes in total body water (TBW) content and its distribution (Fig. 8-1): b. By term, water makes up 75% of body weight and a greater proportion has shifted from ECF to ICF compartments. These changes are largely due to increases in body fat content. 2. Fluid adjustments after birth. b. A physiologic contraction of ECF volume occurs with diuresis in the first week of life, resulting in postnatal weight loss. This is reflected in a weight loss of 5% to 10% in term infants and up to 20% in preterm infants. This may be related to levels of circulating atrial natriuretic peptide (Blackburn, 2013; Modi et al., 2000). B. Regulation of fluid balance. a. Because water and electrolyte balance is regulated by the placenta, the role of the fetal kidneys is primarily to maintain amniotic fluid volume. Fetal nephrons are functional but immature until 34 weeks. Renal blood flow, renal tubular function, and glomerular filtration rate (GFR) are all immature in the fetus and in the extremely premature infant (Kenagy and Vogt, 2013). b. After birth, renal blood flow increases as renal vascular resistance falls. Improved renal function in the days after birth from increased GFR is more pronounced in the term than in the preterm infant. c. Both term and preterm infants can dilute urine; however, when faced with a rapid fluid load, the preterm infant may have a delayed response, resulting in fluid retention. d. Reabsorption of sodium, bicarbonate, and glucose is limited in the newborn infant. 2. Hormonal mechanisms. b. Because of decreased responsiveness to ADH, neonates cannot efficiently concentrate urine in response to fluid deprivation. C. Fluid losses in the neonatal period. 2. Insensible water losses (IWLs): These are the nonmeasurable losses that occur through the skin and respiratory system. Factors influencing IWLs are summarized in Box 8-1. (1) TEWL increases with decreasing gestational age (Lund and Kuller, 2007). (2) TEWL is the major source of IWL in very premature infants. (3) TEWL is highest on the first day after birth, decreasing on subsequent days as the barrier function of the skin improves. This improvement slows with decreasing gestational age, taking several weeks for the development of a fully functional stratum corneum in the extremely premature infant (Lund and Kuller, 2007). (4) TEWL is closely related to ambient relative humidity. TEWL increases with decreasing ambient humidity. (5) TEWL does not appear to be influenced by antenatal steroids or gender (Jain et al., 2000). (6) Failure to account for TEWL increases the possibility of inaccurate estimates of fluid needs, with resultant fluid and electrolyte imbalances. (7) Caring for very premature infants in an incubator capable of providing measured humidity has been shown to decrease TEWL. b. Respiratory losses: roughly 0 to 10 mL/kg/day; related to the temperature and humidity of inspired gases and to minute ventilation. 3. Stool losses: estimated to be 5 mL/kg/day in the first week of life, increasing to 10 mL/kg/day thereafter. 4. Other losses are possible. These include but may not be limited to gastric drainage, enterostomies, surgical wounds, and pleural fluid drainage. D. Fluid therapy. 1. Goal of fluid therapy: The goal is to permit physiologic, adaptive fluid, and electrolyte changes to occur appropriately (Jones et al., 2011). 2. General principles guiding fluid volume decisions. No fixed fluid administration schedules are appropriate for all infants. Lower fluid administration rates are associated with lower incidence of morbidity such as patent ductus arteriosus (PDA) and necrotizing enterocolitis (Bell and Acarregui, 2008). b. Fluid intake is gradually increased on subsequent days to 150 to 175 mL/kg/day, although fluids may be restricted longer for infants with severe cardiorespiratory disorders, renal failure, and postasphyxial syndrome. c. Infants with ongoing fluid losses (chest tube drainage, gastric drainage, enterostomy drainage, diarrhea) may need replacement of these volumes with appropriate fluids. d. It is generally recommended to use birth weight, rather than current body weight, to calculate fluids on a per-kilogram basis until birth weight is regained. 3. Fluid constituents. b. Electrolytes are not usually added to maintenance intravenous (IV) fluids for the first 24 to 48 hours after birth. Serum electrolyte levels and urine output are used to determine when to add these electrolytes to IV fluids. E. Assessment of fluid balance: Quantifying fluid requirements in extremely preterm infants is difficult. Fluid restriction places the infant at risk for dehydration, whereas fluid excess places the infant at risk for intravascular fluid overload (Jones et al., 2011). Close monitoring of hydration status is imperative, with some infants requiring assessment of their fluid balance as often as every 6 to 8 hours. 2. Urine volume: For greatest possible accuracy, urine output must be measured right after it occurs; urine collected onto diapers lying under radiant warmers may evaporate before the diapers are weighed for determination of output. 3. Specific gravity of urine: an indirect measure of urine osmolality. Normal values (1.002 to 1.012) reflect a normal urine osmolality (100 to 300 mOsm/L). Specific gravity is an unreliable predictor of urine osmolality if glucose, blood, or protein is present. 4. Assessment parameters: b. Hemodynamic assessment: pulse quality, blood pressure, and perfusion (capillary refill time, temperature, and acid–base balance). 5. Laboratory evaluation of hydration status: serum sodium level, osmolality, blood urea nitrogen (BUN), creatinine, and/or hematocrit. Disorders of fluid balance in the newborn infant do not always fit neatly into categories such as “fluid depletion” or “fluid excess”; some involve elements of both. One such disorder is septic shock, in which low intravascular volume (a fluid deficit) can coexist with interstitial and cellular edema (a fluid surplus). For simplicity, an attempt is made here to group clinical conditions according to the primary effect on TBW (e.g., decreased, as in dehydration, or increased, as in congestive heart failure), even though there may be an overlap. B. Causes and precipitating factors. 2. Acute blood loss/hypovolemia: hemorrhagic losses at birth, postnatal internal hemorrhage, surgical blood loss, or the removal of large volumes for laboratory tests. 3. Diarrhea. 4. Diabetes insipidus (pure renal water loss from failure to secrete or respond to ADH). This condition is treated with intranasally administered arginine vasopressin (DDAVP). 5. Abdominal or pleural cavity exposure during surgery. 6. Unreplaced losses from gastric suction. 7. Medications that may cause diuresis: caffeine and theophylline. 8. Breastfeeding malnutrition: inadequate intake in a breastfed infant with a cycle of reduced milk production and decreasing demand, resulting in severe malnutrition, dehydration, and hypernatremia. C. Clinical presentation and assessment. 1. Weight loss if net reduction in TBW. 2. Low urine output (< 0.5 mL/kg/hr); possibly high specific gravity. Urine output may be normal or even high in the ELBW infant during postnatal diuresis. 3. Poor skin turgor (gently pinched skin is slow to retract) and dry skin and mucous membranes. 4. Hemodynamic changes: tachycardia or decreased pulses with peripheral vasoconstriction (pale, cool, mottled skin with prolonged capillary filling time), increased core–peripheral temperature differential, central blood pressure either normal or low. 5. In breastfeeding malnutrition, possible excessive sleepiness, disinterest in feeding, or irritability. D. Diagnostic studies. 1. Serum sodium can be low, normal, or high, depending on the cause of dehydration/fluid loss. 2. With dehydration, BUN and creatinine levels may be elevated. 3. Hematocrit levels may be increased or decreased with blood loss. 4. Blood gas values may reveal metabolic acidosis in the infant with hypovolemia. E. Patient care management. 1. Hypovolemic states (shock, hemorrhage) are managed acutely with volume replacement and vasoactive inotropic agents (see Chapter 28). 2. The type of fluid given to replace other fluid deficits depends on the constituents of lost fluid (e.g., free water loss, electrolyte loss) and the infant’s electrolyte levels. Determination of the fluid constitution can be guided by evaluating the electrolyte composition of the fluid being lost. 3. Management of severe dehydration involves replacing the free water deficit slowly over several days to avoid a rapid fall in serum osmolality. F. Fluid management of hypernatremic hyperosmolar dehydration in the preterm infant. 2. The single method or combination of methods most effective in reducing TEWL has yet to be proved. Each of the following strategies will decrease TEWL to some degree. b. Supplemental humidity. Devices to saturate the air immediately surrounding the infant can be used with both incubators and radiant warmers. Humidifier temperature, airflow setting, and seasonal ambient relative humidity variations can significantly affect the achievable humidity level. c. Heat shields or plastic film “blankets” increase ambient humidity by using the infant’s own trapped evaporative losses. d. Semipermeable dressings (adhesive or nonadhesive) may help reduce TEWL. 3. Reduce respiratory water losses by using humidified gas mixtures. 4. Even with maximal reductions in IWL, fluid intake must occasionally be increased, especially in ELBW infants. Giving too much fluid in response to hypernatremic dehydration can aggravate hyperglycemia and increase the risk of heart failure, pulmonary edema, and central nervous system (CNS) injury. It is usually recommended to give just enough fluid to maintain the serum sodium in the high normal range (145 to 150 mEq/L) during the first 24 to 72 hours of life (Jones et al., 2011). 5. Restrict sodium (unless the infant is hyponatremic), adding gradually when serum sodium level decreases and diuresis begins. 6. Monitor hydration closely. Weight loss may be accepted if other parameters indicate adequate hydration. G. Complications. 2. Hypotension, tissue damage, or metabolic acidosis from hypoperfusion. 3. Impaired excretion of drugs when urine output is minimal. 4. Electrolyte imbalances from slow excretion of daily solute load. 5. Renal failure and vascular thrombosis: possible result of severe dehydration. 1. Low colloid osmotic pressure (decreased plasma protein concentration). 2. Increased capillary permeability to water and protein (may be secondary to tissue hypoxia). 3. Increased hydrostatic pressure within the capillaries. 4. Impaired lymphatic drainage of interstitial fluids and proteins. With some of the disorders associated with these pathologic processes, a combination of venous congestion, renal failure, and edema suggests a state of fluid overload even when circulating blood volume is low. B. Etiologies and precipitating factors. 1. Cardiac dysfunction: congenital heart disease, congestive heart failure, PDA. 2. Respiratory distress syndrome and bronchopulmonary dysplasia (BPD). Therapeutic use of oxygen and positive pressure ventilation causes endothelial injury with subsequent fluid leakage. In the first few days after birth, increased lung fluid complicates the picture of respiratory distress and failure to clear the fluid adds to the possibility of developing BPD (Blackburn, 2013; Martin and Crowley, 2013). 3. Perinatal asphyxia. 4. Sepsis, necrotizing enterocolitis. 5. Hydrops fetalis. 6. Renal failure. 7. Miscalculation of fluid needs or provision of too much fluid (possibly from failure to account for all sources of fluid, such as flush solutions, medications, and colloids). 8. Use of neuromuscular blocking agents. 9. Syndrome of inappropriate antidiuretic hormone (SIADH): usually associated with CNS infection or injury. ADH secretion is inappropriate to usual osmotic and volume stimuli. The result is fluid retention with hyponatremia, low serum osmolality, and high urinary sodium loss. C. Clinical presentation and assessment. 1. Weight gain, if there is a net increase in TBW. 2. Urine output: possible decrease. 3. Edema: peripheral, generalized, pulmonary. 4. Hemodynamic changes: dependent on intravascular volume status. When increased, there may be symptomatic PDA, tachycardia, and increased pulses or blood pressure. With congestive heart failure, venous filling pressure is high. D. Diagnostic studies. 1. Serum osmolality is low (< 280 mOsm/L); urine osmolality is normal. 2. In SIADH, osmolalities and sodium levels of urine and serum are diagnostic (urine output is low with high specific gravity and high sodium levels; serum has low sodium level and low osmolality). E. Patient care management: In addition to therapy aimed at the underlying disease process: 2. Diuretics may be useful. 3. Infants with severe edema and low intravascular volume (shock) present a challenge. Maintenance of an adequate circulating blood volume may require volume expansion and vasoactive agents while minimizing maintenance fluid administration. 4. Edema may predispose the infant to necrotic injury of the skin. The skin must be protected from pressure with careful repositioning, support, and the use of a nonrigid sleeping surface, such as a gel- or water-filled mattress. F. Complications. 2. Excessive fluid administration early in life has been associated with worsening of respiratory distress syndrome and development of BPD, symptomatic PDA, and necrotizing enterocolitis. 2. Regulation of Na. Cellular transport of Na is achieved by the sodium–potassium pump, which maintains the electrochemical Na and potassium gradients across the cell membrane. Renal (GFR, tubular function) and hormonal (aldosterone, ADH) mechanisms influence the body content of Na. Although preterm infants can excrete Na, a low GFR early in life may hamper this ability. In addition, minimal responsiveness to aldosterone and ADH contributes to a baseline salt-wasting tendency. 3. Positive Na balance. Na intake greater than Na losses. This is a prerequisite for the growth of new tissue. B. Hyponatremia. 2. Causes and precipitating factors. a. Prematurity (renal and hormonal immaturity, with tendency to excrete Na). Preterm infants are most vulnerable to hyponatremia just after the period of postnatal extracellular volume contraction (Guignard and Sulyok, 2013; Jones et al., 2011). b. Conditions associated with low intravascular volume (e.g., shock). Baroreceptor stimulation of ADH results in reduced renal water excretion and a dilutional hyponatremia. c. Dilutional hyponatremia from excessive free water intake. d. Renal losses related to prematurity or medications (furosemide, methylxanthines). Urine Na excretion rate should be measured to rule out excessive Na losses. e. Inadequate Na intake during period of rapid growth, especially in preterm infants fed exclusively human milk. Called late hyponatremia because it occurs after the first week of life. f. Serum Na can be factitiously low in the presence of hyperlipidemia. 3. Clinical presentation and assessment. b. Infants with late hyponatremia may fail to gain weight. 4. Patient care management. a. Provide Na supplementation after postnatal diuresis begins (usually on day 2). Maintenance Na requirement is 1 to 4 mEq/kg/day and is usually given as sodium chloride (NaCl), though sodium acetate or sodium bicarbonate (NaHCO3), may be used if the infant has metabolic acidosis. In very small infants, early Na supplementation has been associated with increased risk of BPD (Posencheg and Evans, 2013). b. A chronic hyponatremic state is corrected gradually over 48 to 72 hours to prevent injury to brain cells (Posencheg and Evans, 2013). c. Monitor weight, urine output, parameters of hydration, and adequacy of intravascular volume (monitoring of central venous pressure, capillary refill time, and core–peripheral temperature differential). d. When hyponatremia is associated with an excess of body water, fluids are restricted. True SIADH is managed with fluid restriction and monitoring of Na, osmolality, and urine output. e. Commercial preparations designed to fortify human milk supply additional dietary Na for this population. 5. Complications. b. The degree to which the infant’s brain may be able to adapt to chronic hyponatremia is not known; however, chronic hyponatremia does impair skeletal and tissue growth. C. Hypernatremia. 2. Causes and precipitating factors. a. Excessive IWL with insufficient fluid intake (even without added Na). b. High inadvertent Na intake (saline infusions in arterial catheters, NaHCO3, medications) or early addition of maintenance NaCl. c. Breastfeeding malnutrition in term infants. Elevated human milk Na content accompanying insufficient lactation and decreased amount of free water contribute to the hyperosmolar state. d. Diabetes insipidus: deficiency of pituitary-secreted ADH, causing loss of water in excess of loss of Na. 3. Clinical presentation and assessment. a. Signs of dehydration may be present. b. In severe hypernatremia, high-pitched cry, lethargy, irritability, and apnea can progress to seizures and coma. 4. Patient care management. b. Recalculate fluid intake. Fluids may have been restricted too much in light of insensible losses. c. Prevent hypernatremia in ELBW infants. Na supplementation may be withheld longer than usual after birth if serum Na level remains normal. In addition, measures to reduce TEWL will aid in the prevention of hypernatremia (Jones et al., 2011; Posencheg and Evans, 2013). d. The need for saline solutions to maintain catheter patency presents a dilemma. Attempts to lower the infused Na concentration too far result in administration of hypotonic solutions, with risk of hemolysis. 5. Complications: As hypernatremia develops, intracellular water can be drawn out, causing cells to shrink. If this process is rapid, this can affect the brain. Sudden increases in plasma osmolality can also contribute to intraventricular hemorrhage. A. Potassium homeostasis: A generally accepted reference range is 3.5 to 5.5 mEq/L. 2. Regulation: K is distributed both intracellularly and extracellularly. The distribution of K between ICF and ECF is regulated by the sodium–potassium pump and is influenced by acid–base balance, insulin, and glucagon. The excretion of K from the body depends on kidney function, GFR, urine flow rate, and aldosterone sensitivity. B. Hypokalemia: Serum K less than 3.5 mEq/L. 2. Causes and precipitating factors. a. Loss of K in the urine (kaliuresis) during postnatal diuresis, before K supplementation is begun. b. Inadequate K intake. c. Increased gastrointestinal losses from an enterostomy or nasogastric tube output or vomiting. d. Metabolic alkalosis. A high serum pH drives K into cells, resulting in a low serum K. e. Medications including bicarbonate, diuretics, and insulin. Insulin increases cellular uptake of K through stimulation of activity of the sodium–potassium pump. 3. Clinical presentation and assessment: cardiac effects (flattened T waves, prominent U waves, ST segment depression), hypotonia, abdominal distention, and ileus. 4. Patient care management. b. Correction of hypokalemic states must be done cautiously, with continuous cardiac monitoring. 5. Complications. a. Rapid administration of K to correct hypokalemia can lead to fatal arrhythmias. b. Hypokalemia potentiates digitalis toxicity. C. Hyperkalemia: Serum K greater than 6.5 mEq/L. 1. Pathophysiology: Heel-stick samples are often hemolyzed, rendering results unreliable. Venipuncture or arterial line sample must be obtained to determine level. In the ELBW infant, the normal postnatal shift of K from the intracellular to the extracellular compartment is intensified. During the prediuretic phase, this excess K is not efficiently excreted secondary to a low GFR and a low Na excretion rate (Eichenwald, 2013). 2. Causes and precipitating factors. b. Endogenous release of K from tissue destruction, hypoperfusion, hemorrhage, and bruising. c. Metabolic acidosis. A low serum pH shifts K out of cells. d. Renal failure, with decreased K clearance. Tests of renal function: BUN, creatinine should be measured concomitantly. e. Adrenal insufficiency. f. Transfusion with blood stored longer than 3 days. 3. Clinical presentation and assessment: Cardiac effects may be seen—ventricular tachycardia, peaked T wave, or a widened QRS complex. An electrocardiogram should be obtained to detect cardiac arrhythmias. Serum ionized calcium should also be assessed as hypocalcemia may potentiate cardiac toxicity from hyperkalemia. 4. Patient care management. b. Acidosis is corrected. c. Diuretics and low-dose dopamine therapy may improve renal excretion of K. Dopamine also enhances K uptake by stimulation of activity of the sodium–potassium pump. d. Temporary measures may be needed to reduce the effects of circulating K until the total body K level can be reduced. (2) Glucose/insulin infusion to enhance cellular uptake of K. Close monitoring of serum glucose is imperative with this strategy, and the clinician should anticipate the need for increasing the glucose infusion rate. The insulin infusion tubing must be primed to ensure delivery of the drug once the infusion is begun. (3) NaHCO3 (metabolic alkalosis shifts K into cells). e. When other measures fail to normalize K: (1) Cation exchange resins are not the preferred treatment for neonates and should be used only in those infants with refractory hyperkalemia. Oral administration is not recommended in infants. Sodium polystyrene sulfonate (Kayexalate) exchanges Na for K in the intestine to increase the excretion of K. Because the onset of action is within 2 to 24 hours, treatment with this medication alone may not be sufficient to rapidly correct severe hyperkalemia (Taketamo et al., 2012). (2) Exchange transfusion. (3) Peritoneal dialysis or continuous arteriovenous hemofiltration for severe, intractable hyperkalemia. 5. Complications. a. Hyperkalemia is life threatening because of the risk of cardiac arrest. b. Sodium polystyrene sulfonate (Kayexalate) can cause hypocalcemia, hypomagnesemia, and hypernatremia. 2. Regulation: b. Vitamin D acts with PTH to restore Ca to normal levels by increasing absorption of Ca and phosphorus from the intestines and bone. c. Calcitonin, a Ca counterregulatory hormone secreted from thyroid C cells, lowers Ca levels primarily by inhibiting bone resorption. d. Phosphorus (P) also inhibits the absorption of Ca (the higher the P, the lower the absorption of Ca). 3. Serum Ca is transported in three forms: a. Protein-bound Ca, accounting for 40% of total serum Ca. b. Inactivated Ca (complexed with anions such as bicarbonate, lactate, and citrate), accounting for 10% of total serum Ca. c. Free ionized calcium (iCa), the physiologically active form that can cross the cell membrane, accounting for 50% of the total serum Ca. Blood pH influences the amount of iCa: acidosis increases iCa, and alkalosis decreases iCa. B. Fetal Ca metabolism: Fetal Ca needs are met by active transport of Ca across the placenta. Ca accretion increases during the last trimester as Ca is incorporated into newly forming bones. Because maternal PTH and calcitonin do not cross the placenta, the fetus is relatively hypercalcemic, which suppresses fetal PTH and stimulates fetal calcitonin. C. Neonatal Ca metabolism: When the supply of Ca ceases at birth, the neonate depends on stored and dietary Ca to avoid hypocalcemia. After birth, the Ca level declines to its nadir by 24 hours of age, but PTH activity remains low. By 48 to 72 hours, PTH and vitamin D levels rise and the calcitonin level declines, allowing Ca to be mobilized. The serum Ca level returns to normal despite a low Ca intake. Approximately 16% of infants born less than 32 weeks of gestation develop nephrocalcinosis in the face of normal serum Ca levels (Kenagy and Vogt, 2013). Development is multifactorial but is associated with increased furosemide use, increased gentamicin levels, and extreme prematurity. D. Hypocalcemia: Serum Ca less than 7 mg/dL or iCa less than 4.4 mg/dL. 2. Causes and precipitating factors. (2) Infant of a diabetic mother (IDM): prolonged delay in PTH production by infant after birth. (3) Placental insufficiency: reduced Ca stores. (4) Perinatal asphyxia and stress, which precipitate a surge in calcitonin that suppresses Ca. In addition, tissue damage and glycogen breakdown release phosphorus into the circulation, which decreases Ca uptake. (5) Maternal anticonvulsant therapy, which affects hepatic enzymes involved in vitamin D metabolism. (6) Low intake of Ca. (7) Factors that may decrease iCa even when the total serum Ca is normal: exchange transfusion, IV administration of lipid emulsion, alkalosis, or alkali therapy for acidosis. b. “Late” hypocalcemia. (2) Transient congenital hypoparathyroidism or secondary hypoparathyroidism from maternal hyperparathyroidism. An increased PTH level in the mother raises the fetal Ca level and suppresses the fetal parathyroid gland. After birth, the suppressed gland cannot maintain a normal Ca level. (3) DiGeorge syndrome: absence of thymus and parathyroid glands. (4) High-phosphate formulas or cereals. The neonate cannot excrete the excess phosphate; the hyperphosphatemia suppresses Ca. (5) Intestinal malabsorption. 3. Clinical presentation and assessment. b. Severe hypocalcemia (neonatal tetany) is rare and presents with jitteriness, seizures, high-pitched cry, laryngospasm, stridor, and a prolonged Q–T interval. 4. Patient care management. a. Monitor serum Ca of infants at risk: premature, IDM, asphyxiated. b. Early, mild hypocalcemia often resolves without treatment. c. Serious hypocalcemia is treated with boluses and/or continuous infusions of calcium gluconate (can also be given orally). d. Treatment of late hypocalcemia depends on the underlying cause. 5. Complications. b. Tissue necrosis and calcifications can result from extravasated Ca infusions. c. Intestinal necrosis and liver necrosis have been reported with Ca infusion given via incorrectly placed umbilical catheters. E. Metabolic bone disease. 2. Causes and precipitating factors. a. Prematurity: the more immature the infant, the higher the MBD rate. b. Parenteral nutrition: low Ca and P intakes. c. Unsupplemented human milk feeding (inadequate Ca and P content) or use of formulas not designed for the preterm infant. d. BPD secondary to fluid restriction and use of diuretics, with renal Ca wasting. 3. Clinical presentation and assessment. a. MBD is asymptomatic; it is often detected initially on routine x-ray examination. b. Skeletal fractures may be seen in the thoracic cage or extremities. c. Other reported presentation is late-onset respiratory distress from “softening” of the ribs. d. Pain may occur with handling; close monitoring of response is necessary. 4. Diagnostic tests. b. Urine: low or absent P excretion; increased urinary Ca. c. Radiologic bone examinations; wrist x-ray films at age 6 to 8 weeks may be used to monitor for MBD. Early evidence can be difficult to discern because bone mineral content must decrease by 30% to be visible. Photon absorptiometry may be done in centers where the necessary equipment is available. d. X-ray examination; findings may include “washed out” (undermineralized) bones, known as osteopenia, or epiphyseal dysplasia and skeletal deformities, known as rickets (Rubin, 2013). 5. Patient care management and prevention of MBD. a. Maintain Ca/P ratio in parenteral nutrition at 1.3:1 to 1.7:1. b. For enteral feeding, use preterm formulas or human milk supplementation. c. Direct supplementation of Ca and P may be needed. Ca given without P will be inadequately used, resulting in hypercalciuria and possibly nephrocalcinosis. d. Gentle handling of infants at risk and avoidance of chest physiotherapy are warranted to prevent fractures. F. Hypercalcemia: Serum Ca greater than 11 mg/dL or iCa greater than 5.8 mg/dL. 2. Causes and precipitating factors. a. Iatrogenic: overtreatment with Ca or vitamin D. b. Hyperparathyroidism: primary neonatal disorder or secondary to maternal hypoparathyroidism, with chronic stimulation of the fetal parathyroid gland. In hyperparathyroidism the serum Ca level is high, phosphate levels may be low, and urinary Ca and phosphate excretion are high. c. Phosphate depletion: caused by low dietary intake; may be associated with low phosphate content in human milk. d. Subcutaneous fat necrosis: found over the back and limbs; associated with difficult delivery, hypothermia, and maternal diabetes. Pathogenic mechanism is unknown. e. Familial infantile hypercalcemia. f. Hypervitaminosis D: excessive maternal intake of vitamin D. 3. Clinical presentation and assessment. a. Hypotonia, weakness, irritability, and poor feeding, all from a direct effect of Ca on the CNS. b. Bradycardia. c. Constipation. d. Polyuria, dehydration (associated with severe hypercalcemia). 4. Patient care management. a. Hydrate infant and promote excretion of Ca (furosemide has calciuretic action). b. Restrict Ca and vitamin D intake; increase phosphate intake. 5. Complications. a. Nephrocalcinosis from hypercalciuria, but may be seen with normal serum Ca levels. b. Metastatic calcification of damaged cells or tissues throughout the body, including the brain. c. Cardiac effects: bradycardia and arrhythmias. A. Magnesium (Mg) homeostasis. A reference range of 1.5 to 2.5 mg/dL is usually accepted. 2. Fetal and neonatal Mg homeostasis: The fetus receives its supply of Mg by active transport across the placenta. Maternal health and diet can influence the amount of Mg accrued by the fetus. After birth, Mg level falls along with Ca level, then rises to normal within 48 hours. 3. Serum total Mg versus the ionized form: Ionized Mg (iMg) is the biologically active fraction of Mg. Total Mg concentration in the serum does not necessarily reflect iMg activity. 4. Concurrent use of Mg and gentamicin potentiates the neuroblocking effect of the Mg, which may result in apnea. Clinical status must be monitored closely. Slow infusion times for gentamicin are indicated (Taketamo et al., 2012). B. Hypomagnesemia: Serum Mg level less than 1.5 mg/dL. 2. Causes and precipitating factors. b. Increased Mg losses: renal and intestinal disorders, including renal tubular acidosis, diarrhea, short bowel syndrome. c. Endocrine causes: neonatal hypoparathyroidism, maternal hyperparathyroidism. 3. Clinical presentation and assessment. a. Tremors, irritability, and hyperreflexia, progressing to seizures. b. Failure to respond to therapy for hypocalcemia: hypomagnesemia a possibility. 4. Patient care management. b. Seizures are usually unresponsive to anticonvulsant agents. 5. Complications: Overtreatment with magnesium sulfate can result in hypotonia and respiratory depression, hypotension, and cardiac arrhythmias. C. Hypermagnesemia: Serum Mg greater than 2.5 mg/dL. 2. Causes and precipitating factors. a. Excessive Mg load: magnesium sulfate treatment in labor, excess administration of Mg to neonate. b. Reduced excretion of Mg: renal failure, oliguria. 3. Clinical presentation and assessment (may be asymptomatic). a. Respiratory depression, apnea. b. Neuromuscular depression: lethargy, poor suck, loss of reflexes, flaccidity, hypotonia. c. Gastrointestinal hypomotility, abdominal distention. 4. Patient care management. a. Prepare to resuscitate infants born to mothers receiving large doses of magnesium sulfate. b. Hypermagnesemia usually resolves with adequate hydration and urine output. Mg excretion can be increased with furosemide. c. If infant is unresponsive to treatment, exchange transfusion may be necessary. 5. Complications: Cardiac arrest and respiratory failure are possible. B. Buffering system: This is the first line of defense against excess H+ concentration. Buffers, including bicarbonate ( C. Lung regulation: The lungs act to lower the H+ level in the blood by removing CO2, which is produced as a waste product of cellular metabolism. It is then transported to the lungs, where it is removed from the body by ventilation. The rate of CO2 removal can be increased or decreased by altering minute ventilation. D. Kidney regulation: The kidney acts to maintain equilibrium between acids and bases in the body by reabsorbing E. Compensation: When one or more of the body’s regulatory systems fail, other systems have a limited ability to maintain the acid–base equilibrium. When the pH is outside the normal range (< 7.35 or > 7.45), compensation has failed. 2. The lungs attempt to compensate for a metabolic aberration, and the kidneys for a respiratory aberration. The result is a change in pH toward normal despite an abnormal blood PCO2 or Only those disorders classified as primary metabolic problems are discussed here. 1. Pathophysiology: A pH of less than 7.35 or serum 2. Causes and precipitating factors. a. Loss of (1) Prematurity: poor renal conservation of (2) Renal tubular acidosis: decreased proximal reabsorption. (3) Severe diarrhea or ileal drainage. b. Excess acid load: ingestion or endogenous production of acid, greater than the ability to excrete it; increased anion gap. (2) Inborn errors of metabolism: disorders of organic acid and carbohydrate metabolism. (3) Caloric deprivation: catabolism of protein or fat for energy. (4) Parenteral amino acid solutions. (5) “Late metabolic acidosis” of prematurity, caused by intolerance of cow’s milk protein. 3. Clinical presentation and assessment. b. Late metabolic acidosis may present at 1 to 3 weeks of age with poor growth, hyponatremia, and persistent renal acid excretion (urinary pH < 5). Urinary pH greater than 7 with systemic acidosis suggests renal tubular acidosis. c. Infants with profound acidosis (metabolic defects such as congenital lactic acidosis) may have respiratory compensation (tachypnea, hyperpnea) or neurologic depression (seizures, coma) reflecting CNS acidosis. 4. Patient care management. a. Treat the underlying cause of acidosis. b. Correction of severe acidosis (pH < 7.2) is usually with NaHCO3 (concentration of 0.5 mEq/mL), in a 1- to 2-mL/kg dose. Administer slowly (over 1 to 2 hours) by syringe pump or continuous drip; rapid increase in osmolality and pH may be dangerous. c. Late metabolic acidosis, if not self-correcting, is sometimes treated with oral NaHCO3. 5. Complications. b. Impaired surfactant production. c. Electrolyte imbalance: decreased iCa, hyperkalemia. d. Adverse effects of 6. Outcome: In follow-up studies, metabolic acidosis was correlated with poor developmental outcome in VLBW infants (van Alfen-van der Velden et al., 2006). B. Metabolic alkalosis. 2. Causes and precipitating factors. a. Gain of b. Loss of H+ during vomiting or nasogastric suction. c. Increased renal acid loss from diuretic therapy. d. Rapid ECF reduction (contraction alkalosis). 3. Patient care management. a. Decrease NaHCO3 intake if alkali therapy is the cause of alkalosis. b. Restoring fluid and electrolyte balance is critical. 4. Complications: Severe alkalosis causes tissue hypoxia, neurologic damage, and electrolyte disturbances (increased iCa, hypokalemia). (Please refer to Chapter 26 for discussion of respiratory acidosis and respiratory alkalosis.)
Fluid and Electrolyte Management
FLUID BALANCE
Physiologic and Assessment Considerations
DISORDERS OF FLUID BALANCE
Fluid Depletion
Fluid Excess
ELECTROLYTE BALANCE AND DISORDERS
Sodium
Potassium
Calcium
Magnesium
ACID–BASE BALANCE AND DISORDERS
Acid–Base Physiology
), plasma proteins, and hemoglobin, act rapidly to pick up excess H+. The major buffer,
, teams with H+ to form carbonic acid, which dissociates into water and CO2 to be eliminated. The normal
level in the neonate is 22 to 26 mEq/L, lower than in the adult.
and other buffers and by excreting H+ and other acids. In this way, the body eliminates the daily load of nonvolatile acids produced by normal metabolism.
. The lungs compensate much more quickly than the kidneys; however, neither can totally normalize the pH unless the underlying disorder is corrected.
Disorders of Acid–Base Balance
of less than 22 mEq/L can result from the loss of
(buffering capacity) or from excess acid production. The immature kidneys contribute to acidosis by failing both to reabsorb
and to excrete H+ when faced with an acid load. When cells do not receive enough oxygen (because of low blood oxygen levels or diminished perfusion), they must use anaerobic metabolism to meet energy needs. This results in the accumulation in the body of lactic acid (lactate), the level of which reflects the severity of tissue oxygen deficiency. Blood lactate may be a more sensitive indicator of tissue hypoxia than pH and base-excess values (Volpe, 2008). Calculation of the anion gap (difference between positive and negative ions) can be a useful tool to differentiate between excess acid and insufficient
as cause of acidosis. Anion gap = (serum Na+ + K+) − (serum Cl− +
[or serum CO2]). Usual range is 8 to 16 mEq/L. If high (> 20 mEq/L), acidosis is due to excess acid. If normal with elevated chloride level, acidosis is due to loss of
.
: normal anion gap.
.
: cerebral hemorrhage or edema related to wide swings in plasma osmolality. Increased cerebral blood flow, more pronounced when infused rapidly (van Alfen-van der Velden et al., 2006). NaHCO3 can also worsen acidosis by rapidly increasing CO2 if lung disease is present and ventilation is inadequate. NaHCO3 can aggravate hypernatremia and cause tissue injury in extravasation.
from overcorrection of acidosis with NaHCO3.
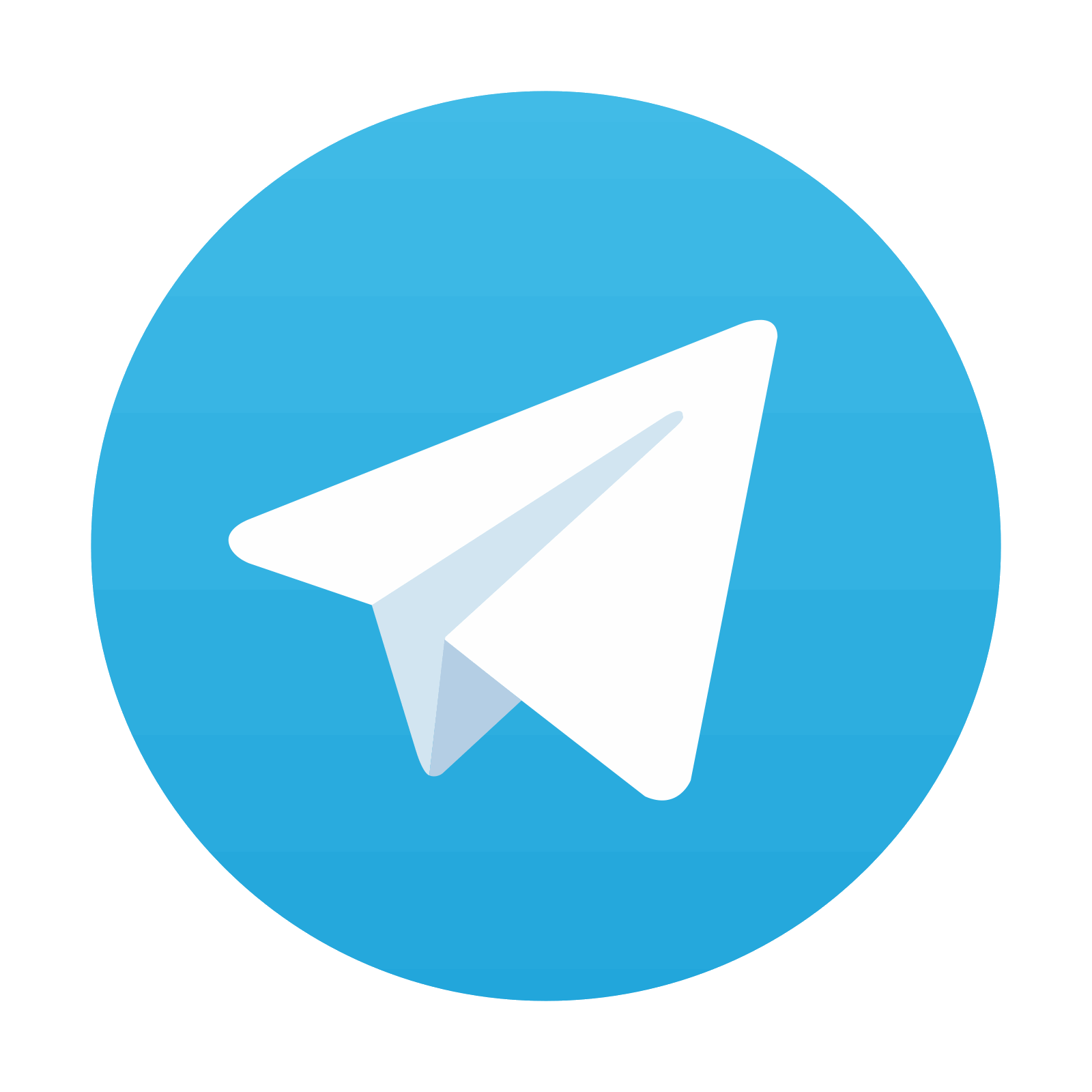
Stay updated, free articles. Join our Telegram channel
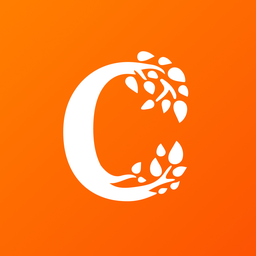
Full access? Get Clinical Tree
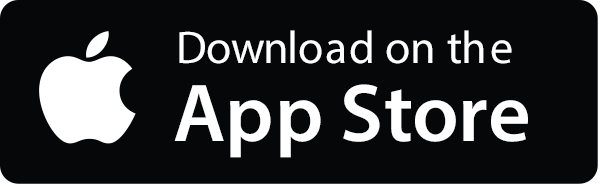
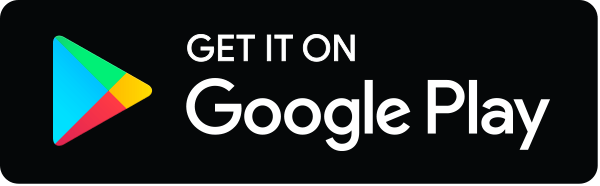