11 Neurologic Disorders
Pearls
• The major goals of therapy in the treatment of traumatic brain injury (TBI) are preservation of cardiopulmonary and cerebral function and the prevention of secondary brain injury.
• The 5 H’s responsible for secondary brain injury in the pediatric patient are: hypotension, hypoxia, hyperthermia, hypo-/hyperglycemia, and hyponatremia.
• Seizures result from abnormal discharges or firing of cerebral neurons that produce alterations in motor function, behavior and consciousness.
• It is important to recognize the difference between posturing and seizure activity. Decorticate and decerebrate posturing typically occur in response to a stimulus, such as a painful stimulus, while seizures can occur at any time and may produce a variety of movements or other evidence of neurologic discharges (e.g., changes in heart rate).
• Decorticate posturing indicates damage along the corticospinal tract, the pathway between the cortex and spinal cord. Decerebrate posturing indicates deterioration of the structures of the nervous system, particularly the upper brain stem; decerebrate posturing has a less favorable prognosis than decorticate posturing.
• Stroke and cerebral vascular disease are among the top 10 causes of childhood death.
• Therapeutic hypothermia has been shown to improve morbidity and mortality following adult cardiac arrest and neonatal hypoxic-ischemic insult. In the pediatric population with TBI, hypothermia remains a controversial therapy. Clinical trials are underway to evaluate the effects of hypothermia in pediatric TBI and hypoxic-ischemic injury.
• When using an extraventricular drainage (EVD) device for CSF diversion, the nurse must maintain the drain at the precise level ordered. If the drain is placed too high, increased ICP may develop before drainage occurs. If the drain is placed too low, excessive drainage of CSF can lead to upward herniation and/or ventricular collapse and intraventricular hemorrhage.
Essential anatomy and physiology
The Axial Skeleton
Blood vessels and cranial nerves enter and leave the skull through small openings, or foramina. It is useful to know the course of the cranial nerves so that clinical signs and symptoms can be correlated with areas of cranial injury (Fig. 11-1). The posterior fossa contains a large foramen, the foramen magnum, through which the brainstem and spinal cord join. Lesions in this area, such as those produced by cervical neck trauma, can interrupt vital brain functions and nerve pathways to and from the brain. Cerebrospinal fluid (CSF) flows through the foramen magnum as it passes from the brain to the spinal cord and back again, and the vertebral arteries enter the skull through the foramen magnum.
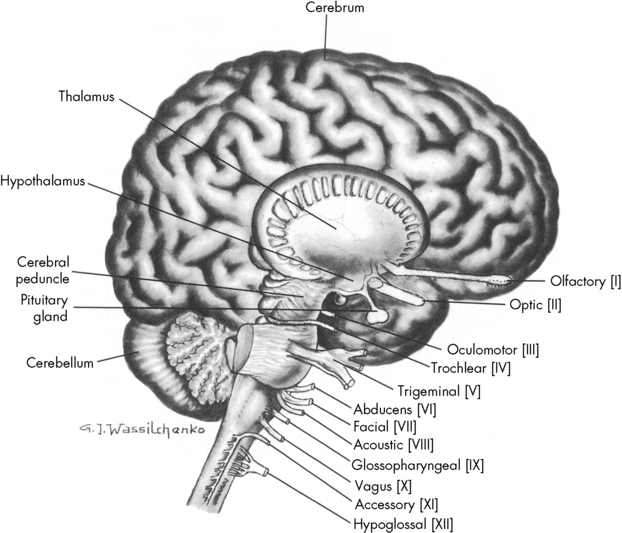
Fig. 11-1 Lateral view of the brain depicting origin of cranial nerves.
(From Rudy EB: Advanced neurological and neurosurgical nursing, St Louis, 1984, Mosby.)
At birth, the skull plates are not fused; they are separated by nonossified spaces called fontanelles. The anterior fontanelle is the junction of the coronal, sagittal, and frontal bones. The posterior fontanelle represents the junction of the parietal and occipital bones (see Evolve Fig. 11-1 in the Chapter 11 Supplement on the Evolve Website). Normally, the posterior fontanelle closes at approximately 2 months of age, and the anterior fontanelle closes at approximately 16 to 18 months of age. If the brain does not grow, such as in patients with microcephaly, the cranial bones can fuse early. Conversely, premature fusion of cranial bones, known as craniosynostosis, can result in microcephaly unless surgery is performed, because brain growth is inhibited by the restricted intracranial space.
At birth, the brain is approximately 25% of the adult volume.76 By 2 years of age, approximately 75% of adult brain volume has been achieved. The cranium itself continues to expand until approximately 7 years of age, when most brain differentiation is complete. This growth of the brain can be assessed indirectly through measurements of the head circumference. These measurements should always be plotted on a growth chart, because they can aid in the detection of excessive or inadequate head and brain growth that may reflect neurologic disease.
The Meninges
Three highly vascular membranes surround the brain and spinal column; the three membranes collectively are called the meninges. The outermost membrane, the dura mater, consists of tough connective tissue that lines the endocranial vault (Fig. 11-2). The dura mater is folded into tents of tissue immediately underneath the skull cap (the periosteum). The most familiar of the many dural folds is the fold that roofs the posterior fossa; this is called the tentorium cerebelli. This fold serves as an anatomic landmark; intracranial lesions are divided into those that occur above the tentorium cerebelli (supratentorial lesions) and those that occur below the tentorium cerebelli (infratentorial lesions). The dura not only lines the endocranium, but it also lines the vertebral column. It descends through the foramen magnum to the level of the second sacral vertebra and ends as a blind sac.
The Brain
The brain is contained within the cranial vault and extends through the foramen magnum. It is composed of distinct structures, each having a specific function. The brain can be considered in three major functional areas: the cerebrum, the brainstem, and the cerebellum (see Fig. 11-1). The cerebrum (from the embryologic forebrain) consists of the cerebral hemispheres, the thalamus, hypothalamus, basal ganglia, and the olfactory and optic nerves. The brainstem consists of the midbrain, pons, and medulla. The cerebellum is the final major division of the brain. The brainstem and cerebellum develop from the embryologic hindbrain. Table 11-1 lists the divisions of the brain and their major functions. Each of these brain divisions is presented separately in the following pages.
Table 11-1 Basic Brain Divisions and Functions
Structure | Division | Function |
Cerebrum | Cerebral hemispheres | Integration of sophisticated sensory and motor activities and thoughts |
Cerebral cortex | ||
Frontal lobes | Reception of smell, memory banks, and higher intellectual processes | |
Parietal lobes | Sensory discrimination, localization of body awareness (spatial relationships), and speech | |
Temporal lobes | Auditory functions and emotional equilibrium | |
Occipital lobes | Vision and memory of events | |
Limbic lobes | Primitive behavior, moods, and instincts | |
Basal ganglia | Transmission of motor tracts, linking pyramidal pathways | |
Corpus callosum | Provision of intricate connection between cerebral hemispheres | |
Brain stem | Midbrain | Hypothalamic response to neuroendocrine stimuli |
Pons | Origin of cranial nerves V, VI, VII, VIII | |
Medulla | Vital center activity (cardiac, vasomotor, respiratory centers); origin for cranial nerves IX, X, XI | |
Cerebellum | White and gray matter | Muscle and proprioceptive activity, balance, and dexterity |
The Cerebrum
The Thalamus and Hypothalamus
The thalamus surrounds the third ventricle and is composed of tracts of gray matter. The thalamus is a major integrating center for afferent impulses from the body to the cerebral cortex.85 The thalamus integrates and modifies messages that come from the basal ganglia and cerebellum and then transmits information up to the cerebral cortex. All sensory impulses, with the exception of those from the olfactory nerve, are received by the thalamus. These impulses are then associated, synthesized, and relayed through thalamocortical tracts to specific cortical areas. The thalamus is the center for the primitive appreciation of pain, temperature, and tactile sensations.
Injury to or disease of the hypothalamus or the pituitary can produce a wide variety of neuroendocrine problems and can result in fluid and electrolyte imbalance and growth disturbances (see Chapter 12).
The Brainstem
The midbrain is a short segment between the hypothalamus and the pons. It contains the cerebral peduncles and the corpus quadrigemina. The midbrain consists of fibers that join the upper and lower brainstem; it is the origin of the oculomotor and trochlear cranial nerves. The midbrain is the center for reticular activity and assimilates all sensory input from the lower neurons before it is relayed to the cortex (see Evolve Fig. 11-2 in the Chapter 11 Supplement on the Evolve Website). It is because of this relay that the cortex can maintain consciousness, arousal, and sleep.
The Cranial Nerves
The cranial nerves are 12 pairs of peripheral nerves that arise from the brain; each has a specific motor or sensory function, and four cranial nerves have parasympathetic functions.111 Cranial nerve function can be lost as a result of lesions near the origin of the cranial nerve or following direct injury to the cranial nerve itself.
Identification of lost cranial nerve functions can help determine the location and severity of CNS disease or injury. For example, pupil inequality and a unilateral sluggish pupil response to light can develop with uncal herniation of the brain (lateral herniation of the temporal lobe through the tentorial notch) and with compression and stretching of the oculomotor nerve. Assessment of cranial nerve function becomes extremely important when the patient is comatose or unresponsive. (Table 11-2 lists cranial nerve origins and functions; see Fig. 11-1).
The Spinal Cord
The spinal cord is a cylindrical structure composed of neurons and nerve fibers. It joins the medulla at the foramen magnum and extends to the level of the second lumbar vertebra. There are 31 pairs of spinal nerves, which are distributed along the entire spinal cord (Fig. 11-3). These spinal nerves are all multifibered and transmit impulses between the CNS and the rest of the body. When a portion of the spinal cord is viewed in cross section, the cord fills only part of the vertebral column; it is surrounded by the pia mater, the CSF, the arachnoid, and the dura mater.
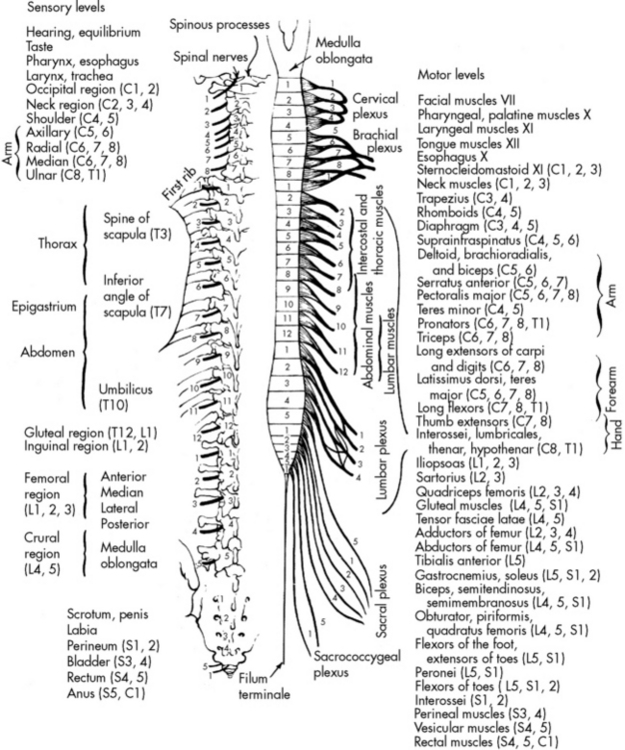
Fig. 11-3 Motor and sensory innervation from the spinal cord.
(From Chusid JG: The spinal nerves. In Feringa ER, editor, Correlative neuroanatomy and functional neurology, ed 18, Los Altos, CA, 1981, Lange Medical Publications.)
Central Nervous System Circulation and Perfusion
The Cerebral Circulation
The brain requires a constant supply of oxygen and substrates (perfusion) to metabolize carbohydrates as an energy source. Adequate perfusion is also necessary to remove carbon dioxide and other metabolites from the brain. The brain requires approximately 20% of the child’s cardiac output. A healthy child’s brain consumes 5.5 mL of oxygen per 100 g of brain tissue per minute.37 As a result, if the brain is deprived of oxygen for even a few minutes, brain ischemia can develop and result in permanent neurologic dysfunction or brain death.32
The cerebral arterial blood flow is provided by the two vertebral arteries and the right and left internal carotid arteries. The internal carotid arteries enter the skull anteriorly and end in the anterior cerebral and the middle cerebral arteries; they supply approximately 85% of cerebral blood flow. The vertebral arteries enter the skull posteriorly and join to form the basilar artery. The basilar artery bifurcates to form two posterior communicating arteries (Fig. 11-4).
The circle of Willis at the base of the brain is formed by a junction of the two internal carotid arteries, the two anterior and two posterior cerebral arteries, and the posterior and anterior communicating arteries (see Fig. 11-4, B). This arterial configuration, present in approximately half of all adults,85 maintains effective cerebral perfusion despite a reduction in flow from any single contributory artery. Patients with an alternative form of arterial circulation are considered to have anomalous cerebral circulation, although their arterial circulation typically is not significantly different from that which is considered normal. Congenital anomalies of one or both carotid arteries or of the internal carotid system have been documented. In many of these patients, the development of collateral circulation early in life prevents any compromise in cerebral perfusion.29
The cerebral venous circulation is unique in that the cerebral veins have no valves, and they do not follow the course of the cerebral arteries.85 Venous drainage from the brain flows primarily into large vascular channels within the dura, known as dural sinuses, that ultimately drain into the internal jugular veins. Occlusion of the jugular vein can obstruct cerebral venous return.
Cerebral Blood Flow and Regulation
Cerebral Blood Flow
Normal CBF in adults is approximately 60 mL per 100 g brain tissue per minute. The normal quantity of CBF in children is unknown, but is thought to be approximately 50 to 100 mL per 100 g brain tissue per minute or more. The absolute quantity of CBF is not as important as the relationship between cerebral oxygen, substrate delivery, and cerebral metabolic requirements. It is essential that CBF is adequate to maintain effective cerebral oxygenation to sustain cerebral functions. Under normal circumstances, CBF, metabolism, and oxygen extraction are closely interrelated.133
Autoregulation
CBF normally is maintained at a constant level by cerebral autoregulation, which is the constant adjustment of the tone and resistance in the cerebral arteries in response to local tissue biochemical changes.72 Autoregulation is essential to the maintenance of cerebral perfusion and function over a wide variety of clinical conditions. If systemic arterial pressure increases, cerebral arterial constriction will prevent a rise in the cerebral arterial pressure to maintain CBF at a constant level. Conversely, if systemic arterial pressures falls, cerebral vasodilation will minimize the effects on CBF. Severe alterations in systemic arterial blood pressure will exceed the limits of autoregulatory compensation, however, and will be associated with changes in CBF.
Autoregulation may be compromised or destroyed with severe traumatic or anoxic brain injury. If cerebral autoregulation is lost, CBF becomes related passively to the mean arterial pressure (MAP) so that a fall in MAP will result in a decrease in cerebral flow and perfusion. Recent studies suggest that impaired autoregulation in traumatic brain injury may correlate with severity of injury and young age.126
Effects of Arterial Blood Gases on Cerebral Blood Flow
CBF is affected by significant changes in arterial oxygen and carbon dioxide tensions.
Carbon Dioxide Response
Normoventilation is the accepted method of management of patients with severe head injury and other causes of increased ICP. Mild hyperventilation to a PaCO2 of 30 to 35 mm Hg should be reserved for acute rises in ICP that are refractory to other medical management (e.g., sedation, hyperosmolar therapy) and that are thought to be associated with acute (impending) herniation syndrome. Although the reduction in CBF caused by hyperventilation can transiently reduce intracranial hypertension, it may worsen ischemia by decreasing oxygen delivery to an already compromised brain.9
When increased ICP is present, routine care such as suctioning must be performed skillfully to prevent the development of hypercarbia and cerebral vasodilation and an increase in ICP.9 The vasoconstrictive response to hypocarbia is unpredictable in patients after traumatic brain injury and the requires careful monitoring of clinical effects of any changes in PaCO2.9
Cerebral Perfusion Pressure
The normal range of CPP is thought to be approximately 50 to 150 mm Hg in healthy adults, with a goal of 70 mm Hg following traumatic brain injury. There is a paucity of information to identify the normal range of CPP in children; it is thought to be approximately 40 to 60 mm Hg, but normal ranges vary with age.9,48 A CPP of at least 40 mm Hg is thought to be necessary for effective cerebral perfusion; however, this number is not absolute because perfusion is determined by blood flow, not blood pressure. It is likely that a CPP of 40 mm Hg is acceptable in an infant, but a CPP of 50 to 65 mm Hg is likely to be necessary in older children and adolescents.
The calculated CPP will fall if the mean systemic arterial pressure falls, if the mean ICP rises, or if both occur simultaneously. The calculated CPP can be maintained despite a rise in ICP if the MAP rises commensurately with a rise in ICP. It is important to note that such compensation may or may not be associated with effective CBF and actual cerebral perfusion (for a Case Study of calculation of CPP, see the Chapter 11 Supplement on the Evolve Website); a normal CPP (40-50 mm Hg or more) has been recorded after brain death was pronounced.16
Evaluation of Cerebral Blood Flow
Jugular Venous Oxygen Saturation
The SjO2 will rise, typically above 75%,127 if oxygen delivery to the brain rises in excess of cerebral oxygen consumption. This rise is unlikely to be caused by decreased cerebral oxygen consumption unless a drug such as a barbiturate is administered. The SjO2 will rise with the development of hypercarbia and associated cerebral vasodilation. An unexpected rise in SjO2 can indicate hyperemia (excessive CBF) that may signal a loss of cerebral autoregulation.
Several calculations can be made using the SjO2 (Box 11-1). Providers can calculate the cerebral extraction of oxygen (normally 20%-42%) and the cerebral arteriovenous oxygen content difference (normally 3.5-8.1 mL/dL of blood).127 A rise in these variables indicates a decrease in oxygen delivery versus demand, with increased oxygen extraction or uptake that may signal decreased CBF. A rise in the arterial-jugular lactate difference can indicate a compromise in CBF.
Box 11-1 Calculations Using Jugular Venous Bulb Oxyhemoglobin Saturation
Cerebral extraction of oxygen (normally 20%-42%):
Cerebral arteriovenous oxygen content difference (cerebral AVjDO2)*:
Note that calculations derived from SjO2 monitoring reflect only global brain oxygenation and cannot identify areas of regional ischemia. In addition, these calculations do not provide absolute values for cerebral metabolic rate and CBF. Sources of inaccurate values include a shift in the oxyhemoglobin dissociation curve—which will change the relationship between oxyhemoglobin saturation and partial pressure of oxygen, thus altering oxygen content at a given saturation—and technical errors related to positioning and calibration, especially in the pediatric population.96
Doppler Flow Velocity
Doppler flow velocity can be measured at the carotid artery or through an open fontanelle. In addition, transcranial Doppler flow velocity studies can be performed in older children and adolescents to evaluate cerebral flow velocity within the middle cerebral artery, anterior cerebral artery, and basal artery. Technical limitations of transcranial Doppler include inadequate visualization through available bone windows, the ability to view only medium to large vessels, variations in measurements between studies, and poor correlation with other indices of CBF.53 There are also limited data regarding pediatric norms. For further information regarding the interpretation of changes in mixed venous oxygen saturations and Doppler flow studies, see the final section of this chapter and Chapters 6 and 8.96
Partial Pressure of Oxygen in Brain Tissue
The cerebral tissue oxygen tension (PbtO2) can be monitored using a probe placed through the skull into brain tissue. The probe can be placed in healthy tissue or in an area of the brain in or near a lesion, to monitor trends in oxygenation. Normal PbtO2 is approximately 20 to 35 mm Hg, and critical values are those less than 15 mm Hg. The PbtO2 can be altered by factors that alter CBF and by those that shift the oxyhemoglobin dissociation curve and alter release of oxygen to the tissues.40
Noninvasive Near-Infrared Spectroscopy
Noninvasive techniques for detection of trends in CBF, including the near-infrared spectroscopy, have been used more frequently in recent years. These techniques require placement of a light source on the scalp to transmit light through the skin and skull into the brain, and then quantify light reflection from the brain. Light reflection will be affected by tissue or hemoglobin oxygenation. These devices can help to identify trends in cerebral perfusion (see Chapter 21).
The Blood-Brain Barrier
Oxygen, carbon dioxide, and lipid-soluble drugs readily cross the blood-brain barrier. The blood-brain barrier is also freely permeable to water, so rapid changes in intravascular osmolality can affect cerebral function (see Chapter 12). The major factor affecting transport across the blood-brain barrier is lipid solubility; the more lipid-soluble the drug is, the more easily it will cross the blood-brain barrier. Many drugs, including some water-soluble contrast agents and some antibiotics, do not cross the blood-brain barrier.
The immature brain does not have adequate development of glial cells; therefore, the blood-brain barrier is incomplete in the preterm infant.64 This incomplete barrier is thought to contribute to increased risk of intracranial hemorrhage in preterm infants. It also makes the neonatal brain more vulnerable to some circulating drugs and toxins.
Cerebrospinal Fluid and Its Circulation
CSF is not merely a filtrate of plasma. It contains water, oxygen, carbon dioxide, sodium, potassium, chloride, glucose, a small amount of protein, and an occasional lymphocyte (Table 11-3). The CSF glucose is normally approximately 75% of the serum glucose concentration, which is approximately 50 to 80 mg/dL. The normal protein concentration is in the range of 20 to 45 mg/dL (higher normal values, up to 125 mg/dL, are present in neonates), and there are usually less than five white blood cells per cubic millimeter present in children. Again, slightly higher numbers may be normal in the neonate.58
Red blood cells are present in a CSF sample only if a traumatic spinal tap was performed or if the patient has suffered a cerebral hemorrhage. Generally, CSF is hypertonic to blood, but changes in CSF osmolality will parallel those of blood (i.e., an increase in serum osmolality will soon be followed by an increase in CSF osmolality). Abnormalities of CSF composition can aid in the diagnosis of some CNS diseases (Table 11-4).
In healthy children, the rate of CSF production is approximately 20 mL per hour.58 The amount of CSF formed is affected by cerebral metabolism, CPP, blood pressure, and changes in the serum osmolality. An increase in the CPP or systemic arterial pressure usually results in an increase in CSF formation.
Once formed, the CSF flows from both lateral ventricles through the foramen of Monro into the third ventricle. From there the fluid passes through the cerebral aqueduct, known as the Sylvian aqueduct (or aqueduct of Sylvius), into the fourth ventricle. Some CSF then passes through the two lateral Luschka’s foramina into the subarachnoid space to bathe the brain. The remaining CSF passes through Magendie’s foramen and enters the subarachnoid space to circulate around the spinal cord. Most CSF ultimately is reabsorbed by venous sinuses that project into the subarachnoid space; these are known as the arachnoid villi (Fig. 11-5). Inflammation (e.g., meningitis) or blood in the ventricular system may obstruct CSF flow, often in the narrow aqueduct of Sylvius between the third and fourth ventricle. Subarachnoid hemorrhage can prevent normal reabsorption of CSF.
Intracranial Pressure and Volume Relationships
Normal Intracranial Pressure
If the brain, cerebral blood, or CSF volume increases without a compensatory decrease in other intracranial components, the intracranial volume increases. Initially, however, the ICP does not rise (Fig. 11-6). This ability to tolerate an increase in the volume of one intracranial component results from the compensatory displacement of venous capacitance blood or CSF from the intracranial vault. In addition, intracranial compliance (including a small amount of brain compression) allows for some increase in intracranial volume without an increase in ICP. However, there is a limit to this compliance. If the brain, blood, or CSF volume continues to increase, ICP ultimately will rise. Once the limits of compliance have been reached, progressively smaller incremental increases in intracranial volume will be associated with progressively more significant increases in ICP (see Common Clinical Conditions, Increased Intracranial Pressure).
Common clinical conditions
Nursing care of any child with an actual or potential neurologic problem requires careful and repeated assessments over time. For this reason, before presentation of common clinical conditions themselves, this section begins with a summary of critical bedside neurologic assessment (Box 11-2).
Box 11-2 Summary of Bedside Nursing Assessment of Neurologic Function
• Airway, Ventilation and Respiratory Pattern, Oxygenation



• Pupil Size and Response to Light: notify on-call provider immediately if pupils dilate or have decreased constriction to light



• Glasgow Coma Scale Score (see Table 11-6)




• Additional Motor Activity and Reflexes

Neurologic assessment and support includes assessment and support of airway, oxygenation, ventilation, and circulation, as well as evaluation of level of consciousness, pupil size and response to light, cranial nerve function, Glasgow Coma Scale score and additional evaluation of motor activity, reflexes, and movement. General neurologic assessment is summarized in Box 11-2. To ensure clear and consistent communication and to facilitate rapid identification of clinical changes, all members of the healthcare team must use consistent terminology and assessment tools and must apply them in a consistent fashion.
Evaluation of Respiratory Pattern
When intracranial injury or insult occurs, some characteristic breathing patterns may be noted that help identify the level of intracranial problem. Such breathing patterns include Cheyne-Stokes breathing, central neurogenic hyperventilation, apneusis, cluster breathing and ataxic breathing (Fig. 11-7). If the ICP rises to a point that brainstem compression occurs and cerebral herniation is imminent, the Cushing reflex is initiated, producing an abnormal breathing pattern that often includes apnea.
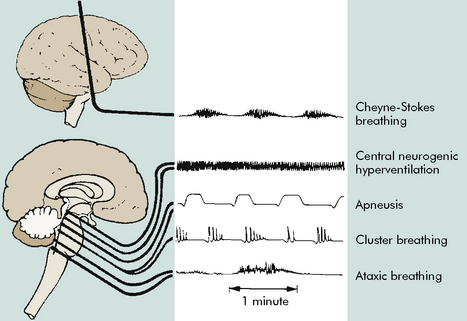
Fig. 11-7 Abnormal respiratory patterns with corresponding level of central nervous system activity.
(From Boss BJ: Alterations in cognitive systems, cerebral hemodynamics, and motor function. In McCance KE, Huether SE, editors: Pathophysiology: the biologic basis for disease in adults and children, ed 6, Philadelphia, 2010, Mosby, p. 531, fig. 16-1.)
Evaluation of Systemic Perfusion
Vital signs are evaluated in light of the patient’s clinical condition (see pages inside front cover for tables of normal heart rates, respiratory rates, and blood pressures in children). Tachycardia and tachypnea are usually more appropriate in critically ill children than are normal heart and respiratory rates. In children who are 1 to 10 years old and of average height, hypotension is present if the child’s systolic blood pressure is less than 70 mm Hg plus twice the patient’s age in years.31,56 Hypotension is also present if the MAP is less than 40 mm Hg plus one and one-half times the child’s age in years.56
The Cushing Reflex
The Cushing reflex is a late and ominous result of increased ICP and ischemia of the vasomotor center.83 The Cushing reflex indicates profound compromise in brainstem perfusion and may develop only when cerebral brainstem herniation is imminent. This reflex produces the clinical triad of bradycardia, an increase in systolic arterial blood pressure with widened pulse pressure, and abnormal breathing pattern. This clinical triad is referred to as the Cushing triad, a term often used interchangeably with the Cushing reflex. The abnormal breathing pattern that is part of the Cushing triad may consist of an abnormal or irregular respiratory effort or apnea.83
Evaluation of Level of Consciousness
If acute coma is present, members of the healthcare team should use consistent terminology (Table 11-5) to describe the child’s level of response. Coma is present when the patient demonstrates no eye opening or verbal response to any stimuli, demonstrating only motor response to painful or noxious stimuli. Stupor is present when only vigorous and repeated stimulation produces arousal.
Table 11-5 Levels of Acute Coma
State | Definition |
Confusion | Loss of ability to think rapidly and clearly; impaired judgment and decision making |
Disorientation | Beginning loss of consciousness; disorientation to time followed by disorientation to place and impaired memory; self-recognition is last to be lost |
Lethargy | Limited spontaneous movement or speech; easy arousal with normal speech or touch; may not be oriented to time, place, or person |
Obtundation | Mild to moderate reduction in arousal (consciousness) with limited response to the environment; falls asleep unless stimulated verbally or tactilely; answers questions with minimal response |
Stupor | A condition of deep sleep or unresponsiveness from which the person may be aroused or caused to open eyes only by vigorous and repeated stimulation; response is often withdrawal or grabbing at stimulus |
Coma | No verbal response to the external environment or to any stimuli; noxious stimuli such as deep pain or suctioning yields motor movement |
Light coma | Associated with purposeful movement on stimulation |
Deep coma | Associated with unresponsiveness or no response to any stimulus |
From Boss BJ: Alterations in cognitive systems, cerebral hemodynamics, and motor function. In McCance KE, Huether SE, editors: Pathophysiology: the biologic basis for disease in adults and children, ed 6, Philadelphia, 2010, Mosby-Elsevier, p. 530, table 16-4.
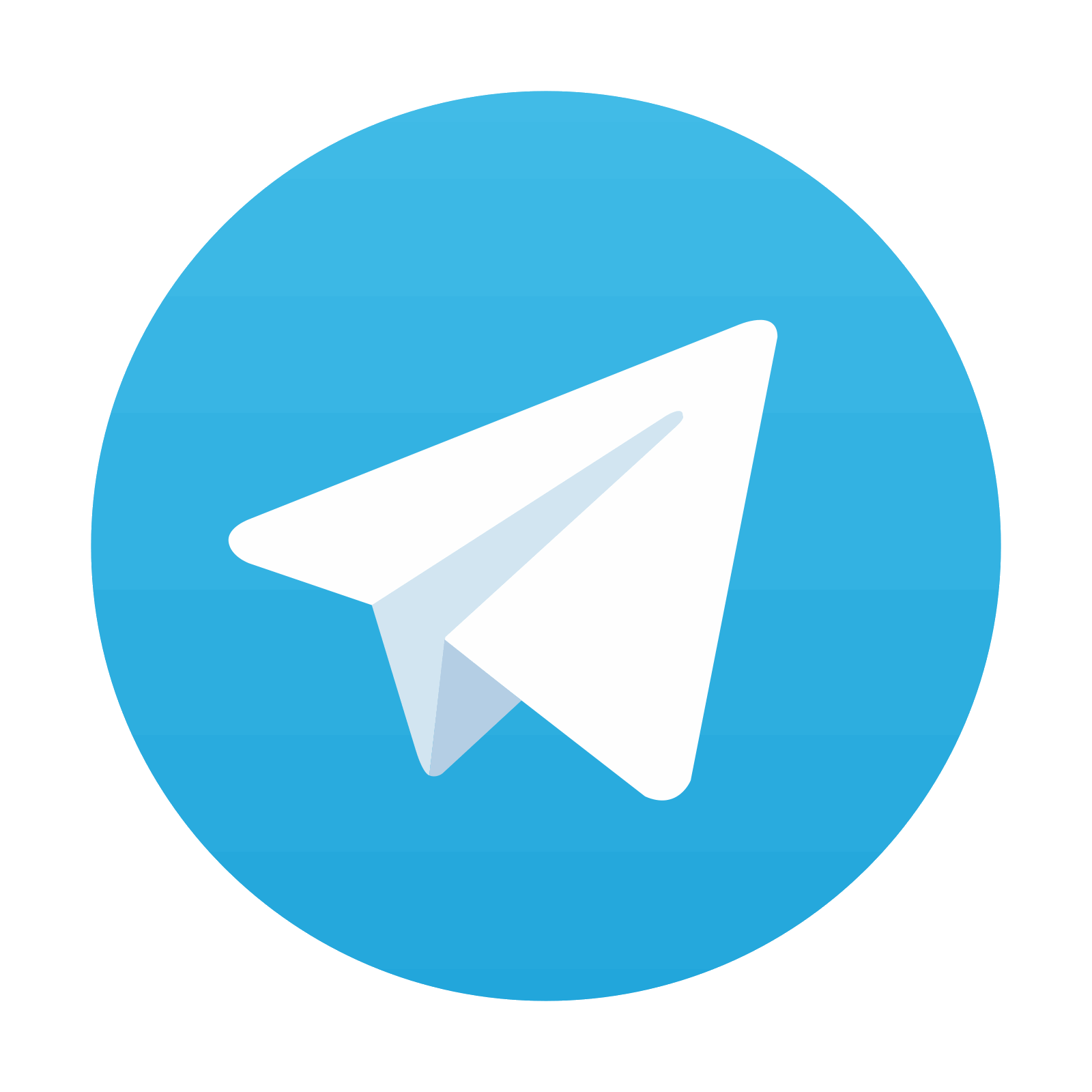
Stay updated, free articles. Join our Telegram channel
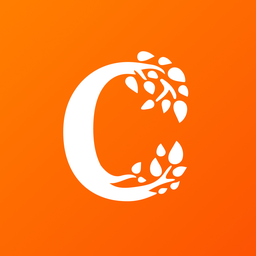
Full access? Get Clinical Tree
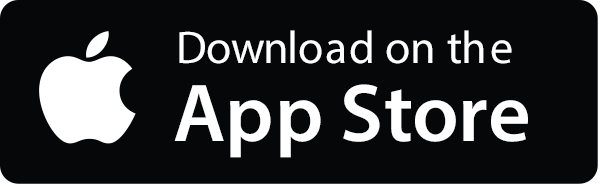
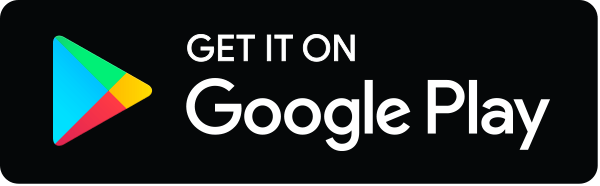