12 The respiratory system
Acidemia: Lower-than-normal blood pH (increased hydrogen ion concentration).
Acidosis: The process that leads to an increase in hydrogen ion concentration in the blood.
Adventitious Sounds: Abnormal noises that may be heard superimposed on a patient’s breath sounds.
Alkalemia: Higher-than-normal blood pH (decreased hydrogen ion concentration).
Alkalosis: The process that leads to a decrease in hydrogen ion concentration in the blood.
Apnea: The absence of breathing.
Apneustic Breathing: Prolonged inspiratory efforts interrupted by occasional expirations.
Atelectasis: Collapse of the alveoli.
Bradypnea: Respiratory rate, in the adult, that is less than 8 breaths/min.
Bronchiectasis: Dilatation of the bronchi.
Bronchospasm: Constriction of the bronchial airways caused by an increase in smooth muscle tone in the airways.
Central Sleep Apnea: A cessation of breathing during sleep as a result of transient abolishment of the drive to the respiratory muscles.
Cheyne-Stokes Respirations: Periods of apnea alternating with rhythmic, shallow, and progressively deeper and then shallower respirations that are associated with brain damage, heart or kidney failure, or drug overdose.
Compliance (Lung): A measure of distensibility of the lungs; the amount of change in volume per change in pressure across the lung.
Cyanosis: A sign of poor oxygen transport, characterized by a bluish discoloration of the skin, produced when more than 5 g of hemoglobin per deciliter of arterial blood is in the deoxygenated, or reduced, state.
Dyspnea: A patient’s perception of shortness of breath.
Epistaxis: Hemorrhage from the nose.
Hypercapnia: Increased tension of carbon dioxide (PaCO2) in the blood.
Hyperoxemia: Increased tension of oxygen (PaO2) in the blood.
Hyperpnea: Increased rate of respirations.
Hyperventilation: Overventilation of the alveoli in relation to the amount of carbon dioxide produced by the body.
Hypocapnia: Decreased PaCO2 in the blood.
Hypoventilation: Underventilation of the alveoli in relation to the amount of carbon dioxide produced by the body.
Hypoxemia: Decreased PaO2 in the blood.
Hypoxia: Inadequate tissue oxygen levels.
Kussmaul Respirations: Rapid, deep respirations associated with diabetic ketoacidosis.
Methemoglobin: Hemoglobin that has the iron atom in the ferric state.
Minute Ventilation (V˙E): The volume of air expired during a period of 1 minute.
Orthopnea: Severe dyspnea that is relieved when the patient elevates the head and chest.
Oxyhemoglobin: Hemoglobin that is fully oxygenated.
Paroxysmal Nocturnal Dyspnea: A sudden onset of severe dyspnea when the patient is lying down.
Partial Pressure: The pressure exerted by each individual gas when mixed in a container with other gases.
Periodic Breathing: A regular waxing and waning of ventilation as a result of fluctuations in central respiratory drive.
Polycythemia: Increased number of red blood cells in the blood.
Rales: Short discontinuous explosive adventitious sounds, usually called crackles.
Reduced Hemoglobin: Hemoglobin in the deoxy state (not fully saturated with oxygen).
Respiration: The process by which oxygen and carbon dioxide are exchanged between the outside atmosphere and the cells in the body.
Rhonchi: Continuous musical adventitious sounds.
Sleep Apnea: Repeated absence of breathing during sleep, sometimes hundreds of times during the night and often for 1 minute or longer.
Torr: Units of the Torricelli scale, the classic mercury scale, which is used to express the same value as millimeters of mercury.
Ventilation: The mechanical movement of air in and out of the lungs.
Wheeze: A high-pitched sibilant rhonchus usually produced on expiration.
Respiratory system anatomy
The nose
The nose, the first area in which inhaled air is filtered (Fig. 12-1), is lined with ciliated epithelium. Cilia move mucus and particles of foreign matter to the pharynx to be expectorated or swallowed (Fig. 12-2). Other functions of the nose include humidification and warming of the inhaled air and the olfactory function of smell.1
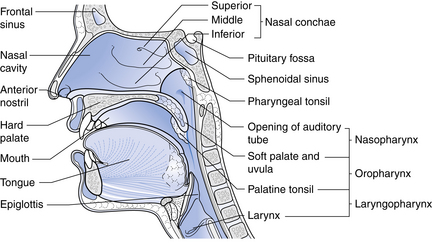
FIG. 12-1 Sagittal section through the nose.
(From Watson R: Anatomy and physiology for nurses, ed 13, Edinburgh, 2011, Baillière Tindall.)
Dry gases are often administered during anesthesia. These gases dry the mucous membranes and slow the action of the cilia. The administration of moist gases in the PACU with various humidification and mist therapy devices keeps this physiologic filter system viable. A tracheostomy precludes the functions of the nose, and proper tracheostomy care, including the administration of humidified oxygen, must be instituted.2
The blood supply to the nose is provided by the internal and external maxillary arteries, which are derived from the external carotid artery, and by branches of the internal carotid arteries. The venous plexus of the nasal mucosa is drained into the common facial vein, the anterior facial vein, the exterior jugular vein, or the ophthalmic vein. A highly vascular plexus of vessels is located in the mucosa of the anterior nasal septum. This plexus is called the Kiesselbach plexus or the Little area. In most instances, this area is the source of epistaxis.3
Epistaxis can occur in the PACU after trauma to the nasal veins from nasotracheal tubes or to nasal airways during anesthesia. If epistaxis occurs, prompt action should be taken to prevent aspiration of blood into the lungs. The patient should be positioned with the head up and flexed forward toward the chest. Cold compresses applied to the bridge of the nose and neck may be effective in slowing or stopping the bleeding. If the bleeding is profuse, the oral cavity should be suctioned carefully and the attending physician should be notified. A nasal pack or cautery with silver nitrate or electric current may be necessary to stop the bleeding.4
The pharynx
The pharynx originates at the posterior aspect of the nasal cavities and is called the nasopharynx until it reaches the soft palate, where it becomes the oropharynx. The oropharynx extends to the level of the hyoid bone, where it becomes the laryngeal pharynx, which extends caudally to below the hyoid bone.1
The larynx
The larynx, or voice box (Fig. 12-3), is situated anterior to the third, fourth, and fifth cervical vertebrae in the adult male. It is situated higher in women and children. Nine cartilages held together with ligaments and intertwined with many small muscles constitute the larynx. The thyroid cartilage, the largest, is V-shaped; its protruding prominence is commonly referred to as the Adam’s apple. The thyroid cartilage is attached to the hyoid bone by the hyothyroid membrane and to the cricoid cartilage. The cricoid cartilage is situated below the thyroid cartilage and anteriorly forms a signet-shaped ring. The signet lies posteriorly as a quadrilateral lamina joined in front by a thin arch. The inner surface of the cricoid cartilage is lined with a mucus membrane. In children younger than 12 years, the cricoid cartilage is the smallest opening to the bronchi of the lungs.
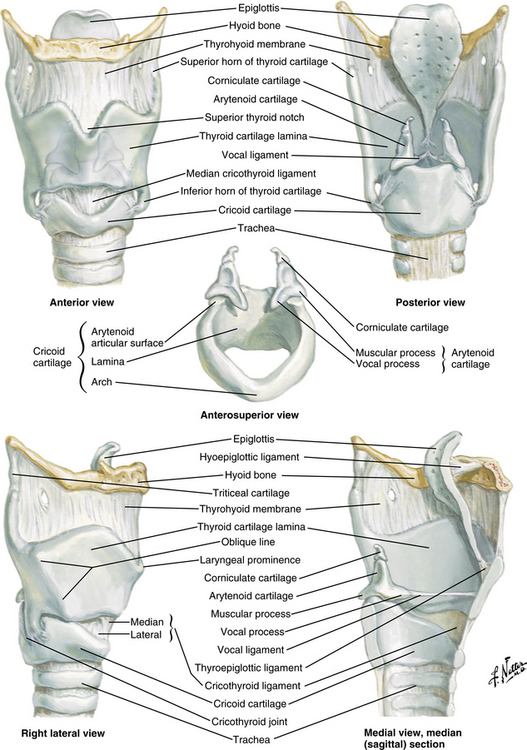
FIG. 12-3 The larynx.
(Netter illustration from www.netterimages.com. © Elsevier Inc. All rights reserved.)
The epiglottis, a cartilage of the larynx, is an important landmark for tracheal intubation that serves to deflect foreign objects away from the trachea. This cartilage is leaf shaped and projects outward above the thyroid cartilage over the entrance to the trachea. The lower portion is attached to the thyroid lamina, and the anterior surface is attached to the hyoid bone and thereby to the base of the tongue. The valleys on either side of the glossoepiglottic fold are termed the valleculae.5
The fissure between the vocal folds, or true cords, is termed the rima glottidis or glottis. In the adult, this opening between the vocal cords is the narrowest part of the laryngeal cavity. Any obstruction in this area leads to death via suffocation if not relieved promptly. The rima glottidis divides the laryngeal cavity into two main compartments: (1) the upper portion is the vestibule, which extends from the laryngeal outlet to the vocal cords and includes the laryngeal sinus, sometimes called the middle compartment; and (2) the lower compartment, which extends from the vocal cords to the lower border of the cricoid cartilage and thereafter, is contiguous with the trachea.6
The muscles of the larynx are also either intrinsic or extrinsic. The intrinsic muscles control the movements of the laryngeal framework. They open the cords on inspiration, close the cords and the laryngeal inlet during swallowing, and alter the tension of the cords during speech. The extrinsic muscles are involved in the movements of the larynx as a whole, such as in swallowing.4
The nerve supply to the larynx is from the superior and recurrent laryngeal nerves of the vagus. The superior laryngeal nerve passes deep to both the internal and the external carotid arteries and divides into a small external branch that supplies the cricothyroid muscles that tense the vocal ligaments. The larger internal branch pierces the thyrohyoid membrane to provide sensory fibers to the mucosa of both sides of the epiglottis and the larynx above the cords.1
The recurrent laryngeal nerve on the right side exits from the vagus as it crosses the right subclavian artery and ascends to the larynx in the groove between the trachea and esophagus (Fig. 12-4). When the nerve reaches the neck, it assumes the same relationships as on the right. This nerve provides the motor function to the intrinsic muscles of the larynx, with the exception of the cricothyroid, and also provides sensory function to the laryngeal mucosa below the vocal cords.
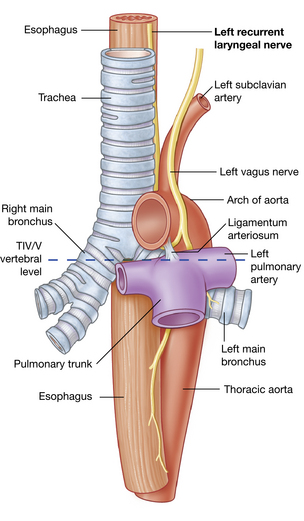
FIG. 12-4 Left recurrent laryngeal nerve passing through the superior mediastinum.
(From Drake RL, et al: Gray’s anatomy for students, ed 2, Philadelphia, 2010, Churchill Livingstone.)
When a laryngospasm occurs in the PACU, prompt emergency treatment is necessary to save the patient’s life. The perianesthesia nurse should have someone on the PACU staff summon the anesthesia provider when laryngospasm is suspected. Treatment consists of mask ventilation with sustained moderate pressure on the reservoir bag. This maneuver usually helps to overcome the partial laryngospasm. Complete laryngospasm not relieved with positive pressure within at least 1 minute necessitates more aggressive treatment. Intravenous (0.5 mg/kg) or intramuscular (1 mg/kg) succinylcholine can be administered to relax the smooth muscle of the larynx. Endotracheal intubation may be necessary.7,8 The nurse must remember that ventilation of the patient’s lungs should be continued until complete respiratory functioning has returned.
The trachea
The area at the distal end of the trachea at the point of bifurcation into the right and left main stem bronchi is called the carina (Fig. 12-5). The carina contains sensitive pressoreceptors, which on stimulation (i.e., with an endotracheal tube) cause the patient to cough and “buck.” The angle created at the point of bifurcation into the right and left main stem bronchi is clinically significant to the perianesthesia nurse. This angle varies according to the age and gender of the patient (Table 12-1). The angle at the right main stem bronchus is smaller than the angle at the left main stem bronchus. Foreign material can easily enter the right main stem bronchus at this point. Endotracheal tubes, if advanced too far, usually enter the right main stem bronchus and thereby occlude the left main stem bronchus. As a result, the left lung cannot be ventilated. Signs of this complication include decreased or absent breath sounds in the left side of the chest, tachycardia, and uneven expansion of the chest on inspiration and expiration.5,9,10
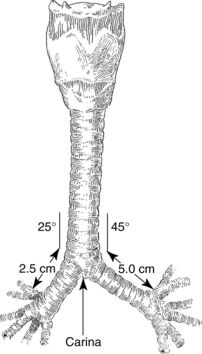
FIG. 12-5 Bifurcation of trachea into main stem bronchi.
(From Nagelhout JJ, Plaus KL: Nurse anesthesia, ed 4, St. Louis, 2010, Saunders.)
Table 12-1 Variations of Bronchial Bifurcation Angles in Adults and Children
RIGHT BRONCHUS (DEGREES) | LEFT BRONCHUS (DEGREES) | |
---|---|---|
Newborn | 12-35 | 30-65 |
Adult male | 20 | 40 |
Adult female | 19 | 51 |
Bronchi and lungs
Each primary bronchus supplies a number of lobar bronchi (Fig. 12-6). Humans have an upper, middle, and lower lobe bronchus on the right and only an upper and lower lobe bronchus on the left. Within each pulmonary lobe, a lobar (secondary) bronchus soon divides into tertiary branches that are remarkably constant as to number and distribution within the lobe. The segment of a lobe aerated by a tertiary bronchus is usually well delineated from adjoining segments by complete planes of connective tissue. These areas of the lung are well defined; therefore pulmonary diseases may be limited to a particular segment or segments of a lobe.
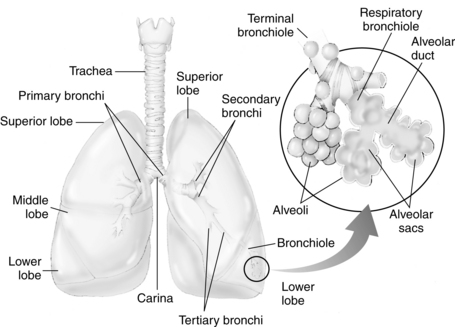
FIG. 12-6 Distribution of bronchi within lungs. Enlarged inset shows detail of alveolus.
(From National Association of Emergency Media: PHTLS prehospital trauma life support , ed 6, St. Louis, 2007, Mosby.)
The terminal bronchioles divide into the respiratory bronchioles in which actual gas exchange first occurs. The respiratory bronchioles bifurcate to form alveolar ducts, and these in turn terminate in spherical enclosures called the alveolar sac. The sacs enclose a small but variable number of terminal alveoli.3
The number of alveoli in an average adult’s lungs is estimated to be 750 million. The surface area available for gas exchange is approximately 125 m2. Alveoli are shaped like soap bubbles in a glass. The interalveolar septum has a supporting latticework composed of elastic collagenous and reticular fibers. The capillaries are incorporated into and supported by the fibrous lattice. The capillary networks in the lungs are the richest in the body.
The lungs receive unoxygenated blood from the left and right pulmonary arteries, which originate from the right ventricle of the heart. The divisions of the pulmonary artery tend to follow the bifurcations of the airway. Typically, two pulmonary veins exit from each lung, and all four veins empty separately into the left atrium. The blood that arrives in the rich pulmonary capillary network from the pulmonary arteries provides for the metabolic needs of the pulmonary parenchyma. Other portions of the lungs, such as the conducting vessels and airways, need their own private circulation. The bronchial arteries, which arise from the aorta, provide the oxygenated blood to the lung tissue. The blood of the bronchial arteries returns to the heart by way of the pulmonary veins.3
Respiratory system physiology
Lung volumes and capacities
Perianesthesia care of the patient is largely based on knowledge of the physiology and pathophysiology of the respiratory system. Dysfunction in lung volumes and capacities that occurs in the patient after surgery is the compelling reason for institution of the stir-up regimen in the PACU. Accordingly, the physiology of the lung volumes and capacities and lung mechanics are described in detail. Table 12-2 provides the definition and normal value for each lung volume and capacity. As shown in Table 12-2 and Fig. 12-7, a lung capacity comprises two or more lung volumes.
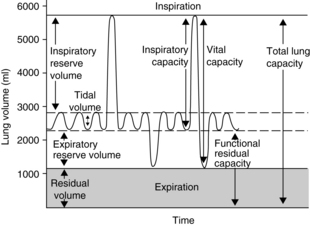
FIG. 12-7 Graphic representation of normal lung volumes and capacities.
(From Hall JE: Guyton and Hall textbook of medical physiology, ed 12, Philadelphia, 2011, Saunders.)
Lung volumes
The expiratory reserve volume (ERV) is the maximal amount of air that can be expired from the resting position after a normal spontaneous expiration. The ERV reflects muscle strength, thoracic mobility, and a balance of forces that determine the resting position of the lungs and chest wall after a normal expiration. This lung volume is usually decreased in patients who are morbidly obese (see Chapter 45). This lung volume also is decreased in the immediate postoperative period in patients who have undergone an upper abdominal or thoracic operation.5,9,10
The residual volume (RV) is the volume of air that remains in the lungs at the end of a maximal expiration. This lung volume represents the balance of forces of the lung elastic forces and thoracic muscle strength. Patients with skeletal muscle relaxant that was not adequately reversed at the end of the anesthetic period may have an elevated RV because enough muscle strength cannot be generated to force all the air out of the lungs. As the RV increases, more air remains in the lungs so that the air does not participate adequately in gas exchange and becomes dead-space air. As the dead-space volume of air increases, it can impinge on the VT and hypoxemia can ensue. The importance of the RV is that it allows for continuous gas exchange throughout the entire breathing cycle by providing air to most of the alveoli and it aerates the blood between breaths. Consequently, the RV prevents wide fluctuations in oxygen and carbon dioxide concentrations during inspiration and expiration.4
Lung capacities
Clinical measurements of the TLC, FRC, and RV are difficult because these values include a gas volume that cannot be exhaled; therefore the measurements require sophisticated pulmonary function testing equipment with gas dilution techniques or plethysmography. Measurements of lung volumes and capacities are useful in the evaluation of lung function.5
Lung mechanics
Mechanical features of the lungs
Mechanical forces of the respiratory system actually determine the lung volumes and capacities. For an understanding of how these lung volumes and capacities are determined and how they are affected by anesthesia and surgery, the perianesthesia nurse should become familiar with the balance-of-forces concept of the respiratory system (see the section on combined mechanical properties of the lungs and chest wall). The PACU stir-up regimen is designed to increase the postoperative patient’s lung volumes and capacities with enhancement of the mechanical forces of the respiratory system.
The lungs and chest wall are viscoelastic structures, one within the other. Because they are elastic, the lungs always want to collapse or recoil to a smaller position. Therefore, as can be seen in the pressure-volume (P-V) curve of the lungs alone (Fig. 12-8), at less than RV the lungs are collapsed and no pressure is transmitted across the lungs (i.e., no transpulmonary pressure). When the lungs are inflated to a volume halfway between RV and TLC, the lungs seek to recoil or collapse back to the resting position equal to or less than RV, which is reflected by an increase in transpulmonary pressure. When the lungs are fully inflated at TLC, a maximal transpulmonary pressure is also exhibited. By analogy, when a balloon is completely deflated, the pressure measured at the mouth of the balloon is zero. When the balloon is partially inflated, the pressure increases as the elastic forces of the balloon try to make the balloon recoil to its resting position. If the balloon is maximally inflated, the elastic recoil of the balloon is greater, as is the pressure measured at the mouth of the balloon.
Pulmonary hysteresis
Inflation and deflation paths of the P-V curve of the lung are not aligned on top of each other (Fig. 12-9). The path of deformation (inspiration) to TLC is different from the path followed when the force is withdrawn (expiration) from TLC to RV. This phenomenon is known as pulmonary hysteresis. The following factors contribute to pulmonary hysteresis: properties of the tissue elements (a minor factor), recruitment of lung units, and the surface tension phenomenon (surfactant).1
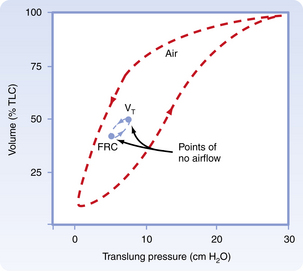
FIG. 12-9 Inflation and deflation paths of pressure-volume curve of lungs.
(From Koeppen BM, Stanton BA: Berne and Levy’s physiology, ed 6 [updated edition], St. Louis, 2010, Mosby.)
Recruitment of lung units
Recruitment of lung units has an important part in pulmonary hysteresis. For an understanding of recruitment of lung units, the nurse must be familiar with the concept of airway closure. An apex-to-base gradient of alveolar size exists in the lung (Fig. 12-10). This gradient occurs because of the weight of the lung, which tends to pull the lung toward its base. As a result, the pleural pressure is more negative at the apex than at the base of the lung. Ultimately, at low lung volumes, the alveoli at the apex are inflated more than the alveoli at the base. At the base of the lungs, some alveoli are closed to ventilation because the weight of the lungs in that area causes the pleural pressure to become positive. Airways open only when the critical opening pressure is achieved during inflation and the lung units peripheral to them are recruited to participate in volume exchange. This process is called radial traction or a tethering effect on airways. An analogy of a nylon stocking can aid in the explanation of this concept. When no traction is applied to the nylon stocking, the holes in the stocking are small. As traction is applied to the stocking from all sides, each nylon filament pulls on the others, which spreads apart all the other filaments; and the holes in the stocking enlarge. Similarly, as one airway opens, it produces radial traction on the next airway and pulls the next airway open; in other words, it recruits airways to open. The volume of air in the alveoli behind the closed airways is termed the closing volume (CV). The CV plus the RV is termed the closing capacity (CC). The CC normally occurs at less than the FRC.5
Surface tension phenomenon
The surface tension phenomenon relates to the action of surfactant on lung tissue. Surfactant is a phospholipid rich in lecithin that is produced by the type II alveolar cells. Surfactant lines the alveolus as a thin surface-active film. This film has a physiologic action of reducing the surface tension of the alveoli and terminal respiratory airways. If the surfactant were not present, the surface tension would be fixed and greater pressure would be needed to keep the alveolus open. As a result, small alveoli would empty into larger ones, atelectasis would regularly occur at low lung volumes, and large expanding pressures would be necessary to reopen collapsed lung units. Surfactant is also an important factor in alveolar inflation because it provides uniformity in the inflation of lung units. In these ways, surfactant helps to impart stability to alveoli in the normal lung. In addition to a major role in pulmonary hysteresis, surfactant also contributes to lung recoil and reduces the workload of breathing.8
Lung compliance
where V represents volume and P represents pressure.
Lung compliance is a measurement of the distensibility of the lungs during breathing. According to convention, CL means the slope on the static deflation portion of the P-V curve over the VT range; therefore CL can be said to be the slope of the P-V curve, and it may remain unchanged even if marked changes in lung elastic properties cause a shift of the P-V curve to the left or right. As a result, clinical measurement of lung compliance is done over the VT range, during deflation. Measurement of the CL over any other portion of the P-V curve can result in an inaccurate reading as compared with a normal value. Lung elastic recoil (PstL) is the pressure exerted by the lung (transpulmonary pressure) because of its tendency to recoil or collapse to a smaller resting state. At low lung volumes, the PstL is low; at high lung volumes, the PstL is high. This elastic retractive force (i.e., PstL) is the result of the overall structural elements of the lung combined with the lung surface tension forces. As mentioned previously, the CL represents the slope of the P-V curve, and the PstL represents the points along the P-V curve. Changes in CL and PstL have dramatic implications in the alteration of lung volumes that occurs in the immediate postoperative period (see the section on postoperative lung volumes).5
Equal pressure point
The alveoli and airways can be imagined as a balloon in a box (Fig. 12-11). Flow out of the balloon is facilitated by the recoil of the balloon, which forces the air out of the balloon through the neck and out into the atmosphere. The addition of pressure all over the box forces the air out of the balloon at a higher rate of flow. Physiologically, the balloon recoil is analogous to the alveolar recoil pressure. The pressure pushing down on the balloon and its neck corresponds to a positive pleural pressure that is generated on a forced expiratory maneuver. For the air to move out of the alveoli, the alveolar pressure must exceed the pressure at the mouth. The pressure inside the neck of the balloon corresponds to the intraluminal airway pressure. Consequently, the alveolar pressure comprises the recoil pressure of the alveoli and the plural pressure. Also, the pressure to generate air flow decreases down the airway to the mouth (see Fig. 12-11). During a forced expiratory maneuver, the plural pressure pushes down on the alveoli and the airways. If the alveolar recoil pressure is 30 and the plural pressure is 20, the alveolar pressure is 50. The pressure inside the airway (intraluminal pressure) decreases progressively downstream toward the mouth. The EPP occurs when the intraluminal pressure is equal to the plural pressure (20 = 20); from that point, the plural pressure exceeds the intraluminal pressure and dynamic compression of the airway occurs. Total collapse of the airways from the EPP and the mouth does not normally occur because of the compliance of the airways. Physiologically, the dynamic compression reduces the airways radius and thus results in an increase in flow rates in the compressed area that aids physiologic mechanisms, such as the cough maneuver, to sheer and expel secretions and mucus out of the airways.5
Pulmonary time constant
The pulmonary time constant is similar to the half-life used in assessment of the pharmacokinetic activity of drugs. A time constant represents the amount of time necessary for flow to decrease by a rate equal to half the initial flow. A time constant equals the resistance multiplied by the compliance. Therefore the time necessary to reach each time constant depends on the individual values of resistance and compliance. In normal conditions, the decrease in flow at the first time constant is approximately 37% of the initial flow, or approximately 63% of the total volume added or removed from the lungs. The first time constant represents the time necessary for removing or adding 63% of the total volume of air in the lungs. The decrease in flow rate at the second time constant is approximately 14%, and the percent volume of air added or removed from the lungs is 86%. The decrease in flow at the third time constant is 5%, with a corresponding 95% volume added or removed; therefore the higher the time constant, the more air removed or added to the lungs.4
Mechanical features of the chest wall
The action of the chest wall can be illustrated with the analogy of a wire screen attached around a balloon. The wire screen tends to spring outward so that the screen pulls the balloon open at lower balloon volumes. A measure of the pressure at the mouth of the balloon reflects a negative number. At approximately 60% of the total capacity of the balloon, the screen no longer tends to spring outward. At that point, the addition of air causes the screen to push down on the balloon—a reflection of a positive pressure at the mouth of the balloon. The screen around the balloon can be likened to the chest wall. As shown in Fig. 12-12, at lower lung volumes, the chest wall clearly is inclined to recoil outward, thus creating a negative pressure. At approximately 60% of the VC, the chest wall starts to push down on the lungs, thus creating a positive pressure. The result of the interplay between the chest wall’s strong tendency to spring outward and the lung’s strong tendency to recoil inward is the subatmospheric pleural pressure.5
Pleural pressure can become positive during a cough or other forced expiratory maneuvers. Pneumothorax can occur when the chest wall is opened or when air is injected into the pleural cavity. With this occurrence, the lungs collapse because they naturally recoil to a smaller position; the ribs flare outward because of their natural inclination to recoil outward. Clinically, inspection of a patient with a pneumothorax may reveal protruding ribs on the affected side.
The two types of pneumothorax are open (simple) and closed (tension). Simple pneumothorax occurs when air flow into the pleural space results in a positive pleural pressure. The lungs collapse because the recoil pressure is not counterbalanced with the negative pleural pressure. Treatment for a pneumothorax can be conservative or more aggressive, depending on the type and amount of pneumothorax. Aggressive treatment consists of the insertion of chest tubes into the pleural space to recreate the negative pleural pressure. This maneuver reestablishes normal ventilatory excursions. In most instances, the air leak between the lung and the pleural space seals after the chest tubes have been removed. If air continues to flow into the intrapleural space but cannot escape, the intrapleural pressure continually increases with each succeeding inspiration. Like a one-way valve, pressure increases and a tension pneumothorax develops. In a brief period, as the intrapleural pressure increases, the affected lung is compressed and puts a great amount of pressure on the mediastinum. Hypoxemia and reduction in cardiac output result, and if treatment is not instituted immediately, the patient may die. Treatment consists of immediate evacuation of the excess air from the intrapleural space with either chest tubes or a large-bore needle. A tension pneumothorax is truly a medical emergency.2
Combined mechanical properties of the lungs and chest wall
The combined P-V characteristics of the lungs and the chest wall have many implications for the perianesthesia nurse. The combined P-V curve is the algebraic sum of the individual P-V curves of the lungs and chest wall. When no muscle forces are applied to the respiratory system, the FRC is determined by a balance of elastic forces between the lungs and the chest wall (Fig. 12-13). Any pathophysiologic or pharmacologic process that affects the elasticity of either the lungs or the chest wall affects the FRC.
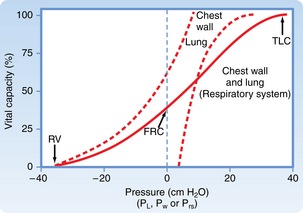
FIG. 12-13 Combined P-V curves of lungs and chest wall. Individual P-V curves of lungs and chest wall are represented with dashed lines. They are transposed from static deflation P-V curves of lungs (see Fig. 12-8) and chest wall (see Fig. 12-12). The combined P-V curve is the algebraic sum of deflation curves of the lungs and chest wall. In a combined P-V curve, FRC can be seen to be determined by balance of elastic forces of the lungs and chest wall when no respiratory muscles are applied. P-V, Pressure-volume; TLC, total lung capacity; RV, residual volume; FRC, functional residual capacity.
(From Koeppen BM, Stanton BA: Berne and Levy’s physiology, ed 6 [updated edition], St. Louis, 2010, Mosby.)
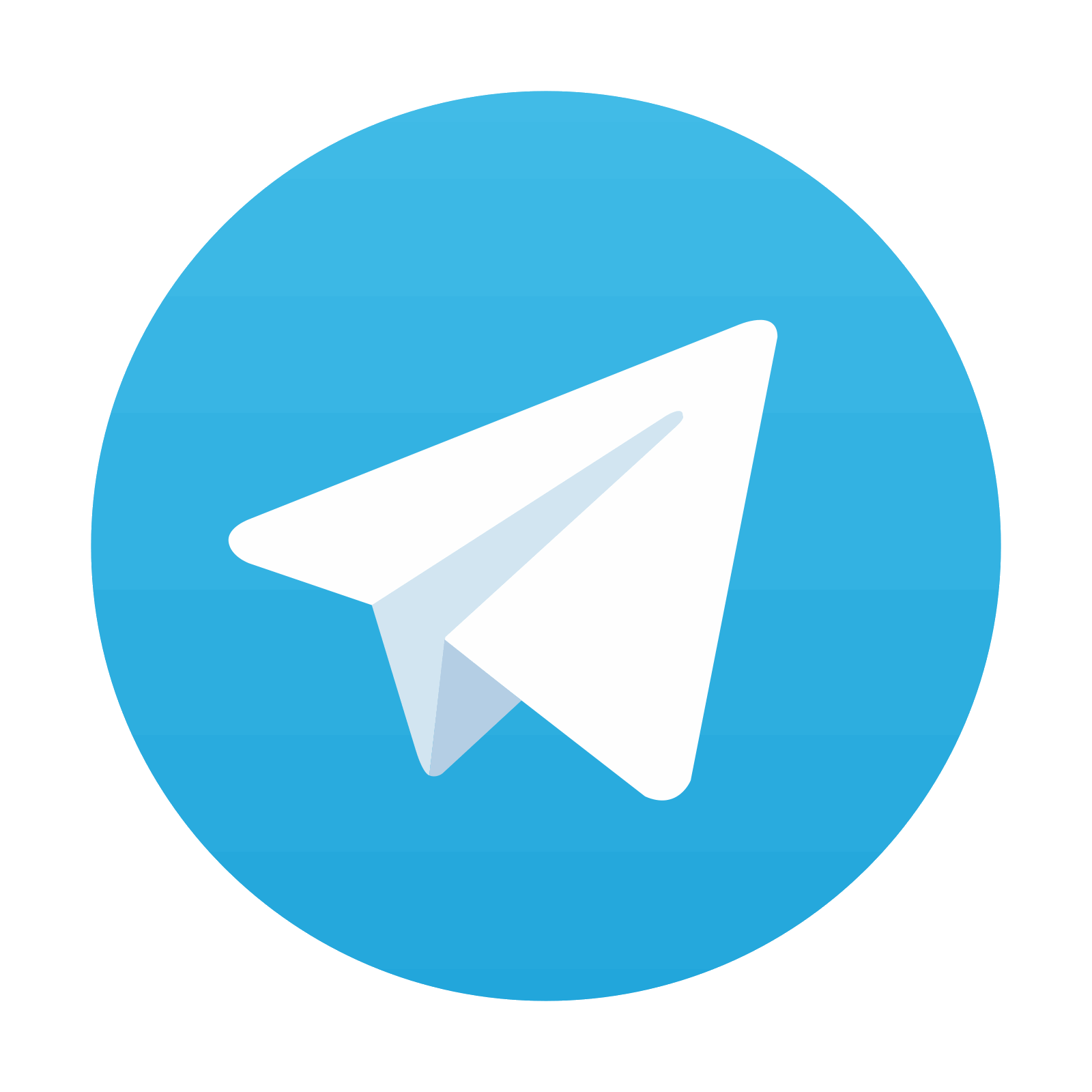
Stay updated, free articles. Join our Telegram channel
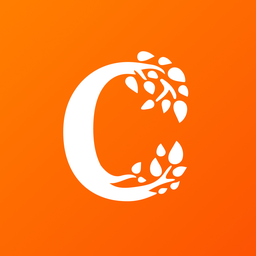
Full access? Get Clinical Tree
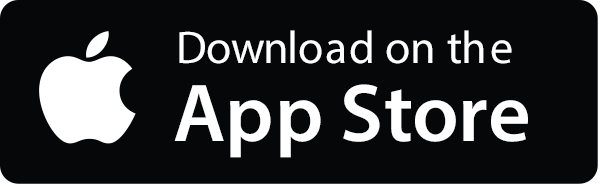
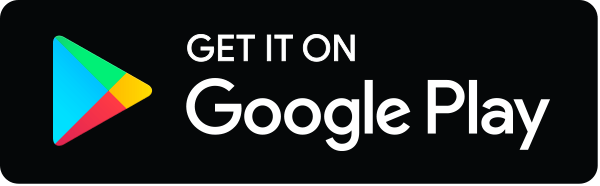