13 Renal Disorders
Pearls
• Urine output will decrease if cardiac output and renal blood flow are compromised; as a result, urine output is a sensitive indicator of cardiac output.
• The normal hourly volume of urine output in children is small, and a small compromise in urine volume may indicate a significant compromise in renal perfusion or function.
• A child’s glomerular filtration rate will approach adult values by about 3 years of age.
• Renal failure may be present in a child with a low, normal, or high volume of urine output.
• Accurate measurement of urine volume and composition provide fundamental data on which clinical decisions are made.
• The modified pRIFLE is a classification system to standardize the definition of acute kidney injury (AKI) in pediatric patients. The acronym, pRIFLE stands for: pediatric risk of renal dysfunction, injury to the kidney, failure of kidney function, loss of kidney function, and end-stage kidney disease. Studies have shown that use of the pRIFLE enhances the classification of AKI epidemiology and the course of AKI in critically ill pediatric patients.
• Renal replacement therapies differ significantly in their effectiveness in treating AKI and its complications. It is therefore helpful for the nurse to be aware of the advantages and disadvantages of each therapy so that the nurse can participate in decisions regarding the best treatment modality for each patient.
Essential anatomy and physiology
Kidney Structure
Gross Anatomy
The kidneys lie anterior and lateral to the twelfth thoracic and first, second, and third lumbar vertebrae and behind the abdominal peritoneum; therefore they are retroperitoneal structures. The kidneys are embedded in a mass of fatty tissue called the adipose capsule, and each capsule is enclosed in the renal fascia (Fig. 13-1). The kidneys are not secured to the abdominal wall, but are held in position by the renal fascia and the large renal arteries and veins. The adipose capsule and the pararenal fat help to protect the kidney and keep it in place.
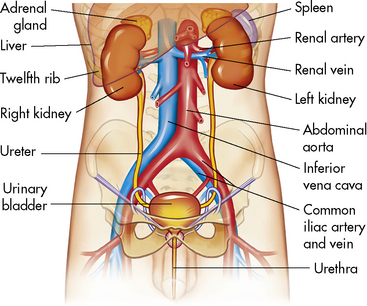
Fig. 13-1 Components of the urinary system.
(From Patton KT, Thibodeau GA: Anatomy and physiology, ed 7. St Louis, 2010, Mosby.)
A longitudinal section of the kidney shows the three general areas of renal structure: the cortex, the medulla, and the pelvis (Fig. 13-2). The renal cortex is the outer portion of the kidney. It has a granular appearance and extends in fingerlike projections into the medullary areas. The cortex contains most of the nephrons, the smallest functioning unit of the kidney. The cortex also contains all glomeruli, the proximal and distal convoluted tubules, and the first parts of the loop of Henle and the collecting ducts.
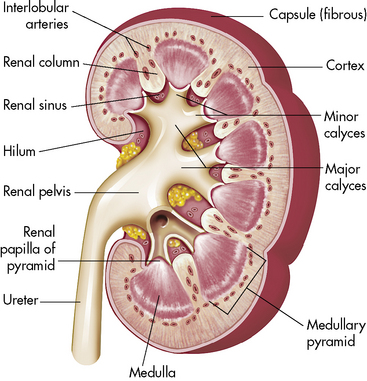
Fig. 13-2 Cross-section of the kidney.
(From Patton KT, Thibodeau GA: Anatomy and physiology, ed 7. St Louis, 2010, Mosby.)
The functioning unit of the kidney is the nephron, which consists of a vascular component and a tubular component (Fig. 13-3). Each kidney contains approximately 1 million distinct nephrons. Eighty-five percent of all nephrons originate in the outermost area of the cortex. The remaining nephrons are the juxtamedullary nephrons that originate in the inner cortical area. The long loops of Henle from the juxtamedullary nephrons that extend deep into the medulla lie parallel to the medullary collecting ducts and play an important role in the concentration of urine (see Evolve Fig 13-1 in the Chapter 13 Supplement on the Evolve Website).
Renal Vasculature
The afferent arteriole enters the glomerular capsule and divides to form the glomerulus, a tuft of capillaries that allows filtration of plasma through the capillary membranes. The glomerular capillaries do not recombine into venous channels, but instead recombine into a second arteriole called the efferent arteriole (Fig. 13-4). Because arterioles are present at either end of the glomerular capillary system, constriction or dilation of these arterioles will alter the resistance to flow through the glomerular capillaries and thus will regulate glomerular filtration.
Renal Tubules and Collecting Ducts
The tubular component of the nephron begins as a single layer of flat epithelial cells surrounding the glomerulus. This layer is known as Bowman’s capsule (Fig. 13-4, B). Filtered plasma from the glomerular capillaries will enter Bowman’s capsule and flow into a coiled tubule called the proximal tubule, also known as the proximal convoluted tubule (Fig. 13-5).
Ureters
The two ureters conduct urine from the renal pelvis to the urinary bladder; they are located behind the peritoneum and they descend through the pelvic cavity, crossing over the common iliac arteries. The ureters are attached to the bladder at an oblique angle; they enter the bladder laterally and tunnel between the bladder mucosa and detrusor muscle, creating a flap-valve design that normally prevents reflux of urine into the ureters during bladder contraction.30
Each ureteral wall has three layers: an inner epithelial lining, a middle muscular layer, and the outer fibrous layer that is continuous with the renal capsule. The middle muscular portion of the ureter consists of both a circular and a longitudinal muscle layer. The circular muscles propel the urine toward the bladder by peristaltic contraction, and they generate enough pressure to overcome the resistance caused by the oblique ureteral insertions into the bladder. Contraction of the longitudinal fibers opens the lumen of the ureter. These ureteral muscle fibers are innervated by fibers from the aortic, spermatic or ovarian, and hypogastric plexuses. Peristalsis persists even when the ureter is denervated, allowing ureters to be transplantable.55
The Bladder and Urethra
The urinary bladder is a hollow, muscular organ that stores urine. There are three openings in the bladder wall: the entrances of the two ureters and the exit of the urethra. These openings form the corners of a triangle, called the trigone. There is a dense area of smooth (involuntary) muscle around the neck of the bladder at the orifice of the urethra; this muscle constitutes the internal sphincter. The urethra extends from the urinary bladder to the body surface. At the point where the urethra passes through the muscles of the pelvic floor, striated (voluntary) circular muscles form an external sphincter.55
If the spinal cord is damaged above the sacral spinal level, the patient initially loses all micturation reflexes because inhibitory and facilitory reflexes from the brain cannot be transmitted through the injured spinal cord. Later, however, simple spinal reflexes can return and the patient can void when bladder distension is sufficient. In this case, the bladder reflex will be initiated at the volume of urine that is usually present in the bladder during the patient’s convalescent period.45
Glomerular Function
Filtration Physiology
Intravascular colloid osmotic pressure, or oncotic pressure, is the pressure opposing free water movement out of the vascular space. It is generated by dissolved proteins, ions, and other particles that are normally present in the blood. Larger particles such as proteins cannot move readily across a capillary membrane; therefore they remain in the vascular space, exerting an osmotic pressure of approximately 35 mm Hg. This oncotic pressure opposes hydrostatic filtration from the vascular space.55
The hydrostatic pressure present in Bowman’s capsule is the pressure exerted on the glomerulus by fluid in the Bowman’s capsule and collecting ducts. This pressure is normally 10 to 15 mm Hg and opposes fluid filtration from the glomerulus. Because tubular fluid is normally protein-free, the oncotic pressure in Bowman’s capsule is normally negligible. (See Evolve Fig. 13-2 in the Chapter 13 Supplement on the Evolve Website for a diagram of these pressures.)
P = mean arterial pressure − venous pressure for that organ
Glomerular Filtration Rate (GFR)
Renal function can be evaluated by calculating the GFR. The GFR, in turn, is roughly equivalent to the creatinine clearance; therefore it can be estimated by calculating the creatinine clearance. This estimate should not, however, be the sole means of determining renal function.56
Creatinine is a small molecule byproduct of skeletal muscle creatine metabolism. Creatinine is released at a near constant rate into the bloodstream, is filtered freely at the glomerulus, and is not broken down, reabsorbed, or synthesized by the renal tubules. Only a tiny amount of creatinine is secreted by the renal tubules. In effect, all of the creatinine that is filtered from the vascular space at the glomerulus remains in the urine and can be measured, and the creatinine clearance mirrors the GFR.62
It is important to note that laboratory determination of serum creatinine concentration may be affected by some cephalosporin antibiotics. For this reason the blood sample for analysis of the serum creatinine level should be obtained when antibiotic drug levels are at their lowest.7
Tubular Function
Reabsorption
Table 13-1 summarizes the work of the renal tubular cells in the process of reabsorption. An average of 180 L of water (protein-free plasma) is filtered through the glomerulus of an adolescent per day, and yet the average urine output is 1.5 L/day. This means that 178.5 L of water is reabsorbed out of the tubular lumen back into the body’s circulation per day.
Table 13-1 Filtration, Excretion, and Reabsorption of Water, Electrolytes, and Solutes by the Kidneys
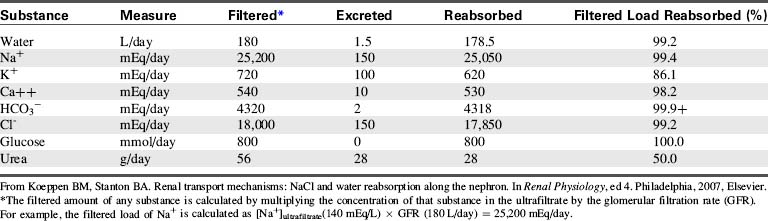
Transport Maximum and Thresholds
If the renal threshold and transport maximum are approximately equal to the daily filtered load of a substance, then the kidneys participate in regulation of the serum concentration of the substance. In such a case, a slight increase or decrease in plasma and filtered concentration of the substance changes its rate of renal reabsorption and excretion, so the serum concentration returns to normal. The renal threshold and transport maximum for phosphate are close to the normal daily filtered load of phosphate, so the serum phosphate concentration is regulated by kidney tubular function. Phosphate transport and reabsorption also will be affected by the serum calcium concentration, parathyroid hormone (PTH), and adrenal cortical hormones.63
Reabsorption and Secretion in the Proximal Tubule
The selective reabsorption of solute begins in the proximal tubules. Approximately 67% of the filtered water, Na+, Cl−, K+, and other solutes such as bicarbonate are reabsorbed in the proximal tubule. In addition, the proximal tubules normally reabsorb all filtered amino acids and glucose.63
Sodium
The primary mechanism for regulation of intracellular and extracellular fluid volume involves renal sodium excretion.63 Sodium is filtered freely at the glomerulus, so its concentration in the proximal glomerular filtrate is identical to its plasma concentration. Sodium is reabsorbed by an active transport mechanism; the mechanism is carrier-mediated and requires energy so the sodium can move against a gradient. Sodium is not secreted into the tubules.
Bicarbonate and Hydrogen Ions
As a result of this process, for every bicarbonate ion that combines with a hydrogen ion in the lumen of the tubule, a bicarbonate ion ultimately will diffuse into the peritubular capillaries (Fig. 13-6). This secretion of hydrogen ions and reabsorption of bicarbonate ions occurs along the length of the renal tubules, but 90% of bicarbonate reabsorption occurs in the proximal tubule. (See section, Regulation of Acid-Base Balance.)
Potassium
Nearly all filtered potassium is reabsorbed by the proximal tubule, and the remaining potassium is reabsorbed in the ascending limb. The proximal reabsorption of the filtered potassium occurs at a constant rate and does not alter, despite the presence of hyperkalemia or hypokalemia. Potassium is also secreted by the distal convoluted tubule and cortical collecting duct, and the rate of K+ reabsorption or secretion in these tubular segments depends on a variety of hormones and factors. Overall, the rate of renal K+ excretion is determined by the distal convoluted tubule and the cortical collecting duct.63 The net result is normally a continuous loss of potassium in the urine.
Calcium
Calcium reabsorption is controlled by parathyroid hormone. Just as for sodium reabsorption, 80% to 90% of filtered calcium is reabsorbed in the proximal tubule and loop of Henle; only 10% of filtered calcium enters the distal tubule for concentration adjustments. When sodium reabsorption is inhibited by loop diuretics, calcium excretion is enhanced.79 Thiazide diuretics increase calcium reabsorption.
Drugs
The glomerulus is nonselective in its filtration of solutes, because the glomerular membrane does not restrict the passage of small molecules. Most drugs are of a small molecular size, and only a fraction of any drug is bound to serum albumin; most will filter into the tubular fluid. Changes in the GFR or in the degree of protein binding will alter the amount of drug present in the glomerular filtrate. Protein binding of a drug can be influenced by competition between drugs for the same protein binding sites (see Chapter 4).
The Loop of Henle
The loop of Henle, located within the renal cortex and the medulla, provides a countercurrent mechanism for urine concentration. The descending limb of the loop of Henle does not transport sodium or chloride actively, but it is highly permeable to sodium and water. Thus, as the filtrate passes through the descending limb of the loop, it becomes progressively more concentrated. The osmolality can increase from 300 to 1200 mOsm/L between the beginning of the descending limb and the tip of the loop of Henle (Fig. 13-7).
The blood vessels surrounding the loop of Henle form a hairpin loop structure, called the vasa recta. The vasa recta consists of capillaries that run parallel to the loop of Henle and the collecting ducts (for an illustration, see Evolve Fig. 13-1 in the Chapter 13 Supplement on the Evolve Website). As these capillaries follow the loop of Henle into the interstitium of the renal medulla, where osmolality is high (as the result of the tubular countercurrent mechanism), water shifts out of capillaries into the interstitial fluid, and sodium and chloride move from the interstitial fluid into the capillaries.
The Distal Tubule and Collecting Ducts
The distal tubule arises from the ascending limb of the loop of Henle; its thick cellular structure is distinct from the thin cells of the ascending loop. Thick cuboidal cells continue up through the renal cortical area to a point where the distal tubule is in direct contact with the afferent arteriole of its glomerulus. At this junction, the distal tubule cells become more densely packed and more columnar, and the muscle cells of the arteriole enlarge and take on a granular appearance. This point of contact between the distal tubule and the glomerular afferent arteriole is called the juxtaglomerular apparatus (see Fig. 13-3).
Renin, Aldosterone, and Antidiuretic Hormone
Renin is secreted from the polkissen cells of the afferent arteriole in the juxtaglomerular apparatus. In turn, renin forms angiotensin I from renin substrate (a circulating peptide from the liver). The amounts of renin released and angiotensin formed are determined by the renal perfusion pressure, sympathetic nervous system stimulation, circulating vasoactive substances, and changes in electrolyte concentration.55
Angiotensin I circulates to the lung and is converted enzymatically to angiotensin II. Angiotensin II produces peripheral vasoconstriction and an increase in aldosterone secretion, which increases renal sodium and water reabsorption. These effects should increase intravascular volume (Fig. 13-8). Angiotensin I and II are destroyed by angiotensinase, an enzyme that is present in plasma and secreted by a variety of organs, such as the kidney, intestine, and liver.
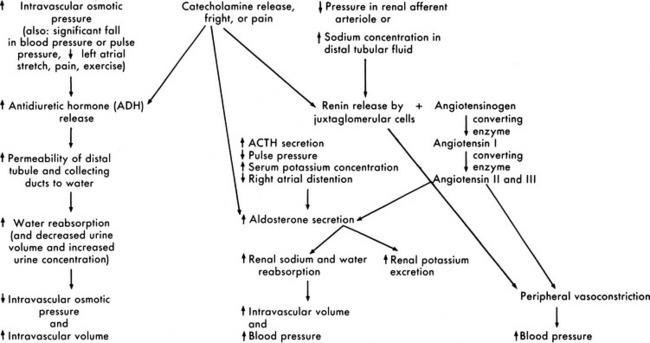
Fig. 13-8 Renal response to changes in extracellular fluid volume and electrolyte concentration or stress.
Aldosterone is secreted by the adrenal cortex in response to pituitary adrenal corticotropic hormone (ACTH) secretion and a variety of other stimuli. A fall in the pulse pressure, decreased stretch of the right atrium, and an increased serum potassium concentration all stimulate aldosterone secretion.63 An important stimulus for aldosterone is formation of angiotensin from renin released by the juxtaglomerular apparatus. Aldosterone stimulates epithelial cell transport of sodium in the renal tubular epithelium, along the intestinal lumen, and in sweat and saliva. Increased aldosterone levels increase the active reabsorption of sodium and decrease potassium reabsorption. The increased sodium reabsorption produces water reabsorption; this increases intravascular volume and reduces the juxtamedullary secretion of renin. The reduction in potassium tubular reabsorption increases potassium excretion in the urine and should result in a fall in the serum potassium concentration. These responses to aldosterone should in turn reduce the stimulus for aldosterone secretion (see Fig. 13-8).
Antidiuretic hormone (ADH), or arginine vasopressin (AVP), secretion also affects the final concentration of urine. ADH is produced by the supraoptic and paraventricular nuclei in the hypothalamus and is transported to the posterior lobe of the pituitary, where it is released in response to an increase in serum osmolality. ADH secretion is stimulated by serum osmolality greater than 280 to 285 mOsm/L (or a rise in serum osmolality of 2% or more). It also is secreted in response to significant (10%-15%) volume depletion, a fall in blood pressure, painful stimuli, fear, and exercise. Hemoconcentration, diabetic ketoacidosis,90 and mannitol administration increase ADH secretion, and administration of hypertonic glucose often inhibits ADH secretion.34,54 The predominant stimulus for ADH secretion is a rise in serum osmolality sensed by osmoreceptors in and around the supraoptic nucleus of the hypothalamus.
Regulation of Acid-Base Balance
Buffering Systems
The Bicarbonate-Carbonic Acid Buffering System
The increase in hydrogen ion concentration will result in a fall in serum pH unless or until CO2 elimination by the lungs is enhanced and/or hydrogen ion excretion and bicarbonate ion reabsorption by the kidneys is increased (see section, Interpretation of Blood Gas Values).
Additional Plasma Buffers
Proteins present in the blood can also act as buffers. Hemoglobin is the most important nonbicarbonate buffer. It binds with hydrogen ions and transports CO2 from the tissues to the lungs for elimination.34
Renal Hydrogen Ion Excretion and Bicarbonate Reabsorption
New bicarbonate can be formed when CO2 combines with water, yielding carbonic acid. The carbonic acid then dissociates into hydrogen ions and bicarbonate; the hydrogen ion is bound to phosphate buffers or ammonia to form hydrogen phosphate or ammonium (). Hydrogen phosphate and ammonium are nonreabsorbable, and they are excreted unchanged in the urine. When hydrogen ions are excreted in this way, a quantity of acid can be measured in the urine; this buffering mechanism results in the formation of titratable acids (see Fig. 13-6). The amount of hydrogen ion excreted in the urine is limited, because the kidney cannot secrete urine with a pH lower than approximately 4.4. In addition, the formation of titratable acid will be limited by the amount of ammonia, phosphate, and other inorganic buffers available.
Interpretation of Blood Gas Values
Evaluation of the pH and PaCO2
Blood gas analysis requires evaluation of the pH, the PaCO2, the calculated base deficit or excess, and the serum bicarbonate. The first step is evaluation of the pH. If the pH is less than 7.35, acidosis is present; if the pH is greater than 7.45, alkalosis is present. The second step is evaluation of the PaCO2 in light of the pH to determine whether any existing change in pH can be explained by the alteration in PaCO2. For every uncompensated torr unit rise in PaCO2 above 45, the pH should fall 0.008 units below 7.35, and for every uncompensated torr unit fall in PaCO2 below 35, the pH should rise 0.008 units above 7.45. Acidosis or alkalosis in excess of that predicted from the PaCO2 must be metabolic in origin (Box 13-1).
Box 13-1 Evaluation of pH and PaCO2 and Calculation of Base Deficit or Excess
1. Subtract the child’s PaCO2 from 45 (result can be a negative or positive number)
2. Multiply difference obtained in Step 1 by 0.008 (result can be a negative or positive number).
3. Add the product obtained in Step 2 and 7.35; this yields the pH predicted from the PaCO2 alone. If child’s pH is lower than predicted, metabolic acidosis is present; if the pH is higher than predicted from the PaCO2, metabolic alkalosis is present.
4. To calculate base deficit, subtract the predicted pH (calculated in Step 3) from the child’s actual pH and multiply this difference by 0.66. A base deficit more negative than (larger than) −2 indicates the presence of metabolic acidosis, and a base excess greater than 2 indicates the presence of metabolic alkalosis.
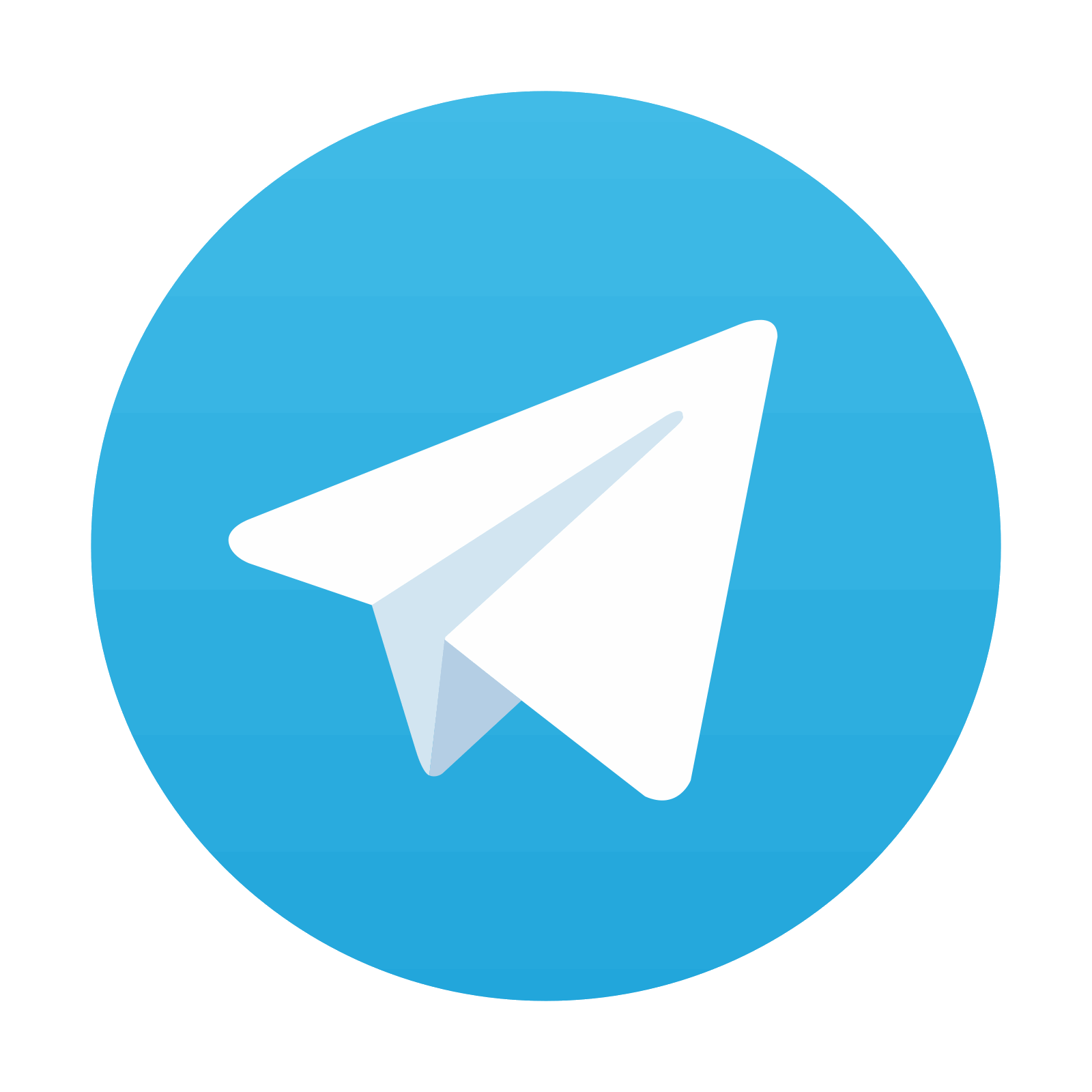
Stay updated, free articles. Join our Telegram channel
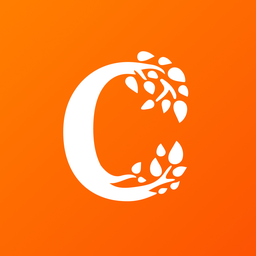
Full access? Get Clinical Tree
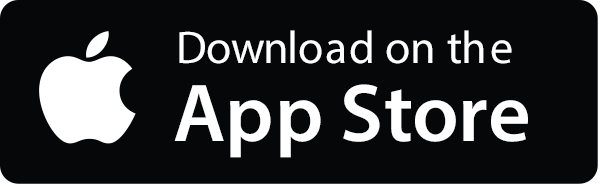
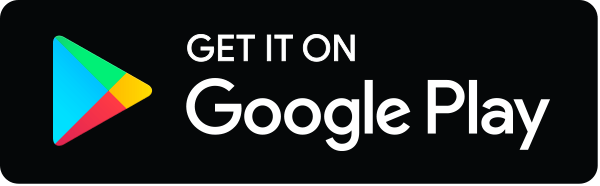
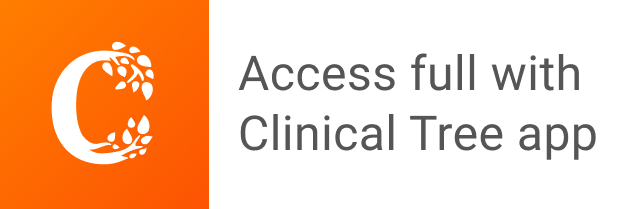