24 Local anesthetics
Conduction Block Anesthesia: Local anesthetic injected in the immediate vicinity of a major nerve plexus (brachial plexus, lumbar plexus, and neuraxial anesthesia).
Field Block Anesthesia: Local anesthetic injected, in a fanlike manner, into tissue surrounding an incision or puncture site.
Infiltration Anesthesia: Local anesthetic injected at an incision or puncture site.
Intravenous Anesthesia: Lidocaine is injected into the vein of an exsanguinated extremity with an inflated tourniquet.
Neuraxial Blockade: A generic term that encompasses both spinal and epidural anesthesia.
Peripheral Nerve Block: Local anesthetic deposited in the immediate vicinity of an individual nerve to produce anesthesia.
Topical Anesthesia: Local anesthetic applied to skin or mucous membranes (pharyngeal cavity or urethra). Systemic absorption from the mucous membranes is rapid. Excessive dosing can lead to toxicity.3–5
Local anesthetics are used to render a portion of the body insensate to painful stimuli through reversible nerve conduction blockade. Erythroxylon coca, the source of cocaine, was used in antiquity. In 1855, cocaine was isolated from the coca bean and rapidly found a place in modern medicine. In 1884, Carl Koller demonstrated its ability to anesthetize the eye, and William Halsted used cocaine for infiltration and nerve blocks. Cocaine had two major problems: toxicity and physical dependence. The first ester local anesthetic suitable for injection, procaine, was introduced in 1905. In 1948, Lofgren introduced the first clinically useful amide local anesthetic.1,2 The use of local anesthetics for anesthesia and/or postoperative analgesia continues evolve. Advances in pharmacology and technology have increased the use of local anesthetics. Perianesthesia nurses should understand the basic physiology of nerve conduction, pharmacology of local anesthetics, and identification and treatment of complications that may arise from their use.
Nerve physiology and conduction
Neurons convey information to and from the central nervous system (CNS). Sensory information is transmitted from the periphery by afferent neurons to the CNS. Motor impulses are transmitted by efferent neurons from the CNS to the periphery. Individual neurons contain a cell body, dendrites, and axons. Dendrites are multiple extensions of the cell body that transmit information toward the cell body. An axon is a single extension that transmits information away from the cell body. Axons are enveloped in a cell membrane known as the axolemma. Endoneurium is the connective tissue that envelops individual nerve fibers. Several nerve fibers form fascicles and are in turn covered with perineurium. Several fascicles covered with perineurium are bundled together and covered by connective tissue to form the epineurium that encompasses the entire nerve (Fig. 24-1).4–7
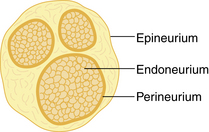
FIG. 24-1 Transverse section of a peripheral nerve.
(From Miller RD, et al: Miller’s anesthesia, ed 7, Philadelphia, 2009, Churchill Livingstone.)
Neurons contain positive and negative charges. At a resting state, the inside of the cell is more negative than the more positive extracellular charge. Within the neuron there are potassium (K+) ions, which have positive charges (cations), and proteins, which have negative charges (anions). The negatively charged proteins are impermeable to the walls of the cell, whereas the positively charged K+ ions are small enough to diffuse through small openings. Because negative ions attract positive ions, there is an accumulation of K+ within the neuron. The ratio of intracellular K+ to extracellular K+ is approximately 30:1. Extracellularly, sodium (Na+) is found in abundance. The ratio of extracellular Na+ to intracellular Na+ is approximately 10:1. Sodium ions are less permeable to the cell membrane and gain access through passive diffusion. Sodium specific channels are closed at this time, so the amount of Na+ that reaches the cell is low. In addition, the NA+/K+ pump actively maintains the gradient between extracellular Na+ and intracellular potassium. For every three Na+ ions that are removed from inside the cell, two K+ ions are replaced (Fig. 24-2). The net difference between the intracellular K+ (negative) and extracellular Na+ (positive) results in a resting membrane potential of –70 to –90 millivolts.4,7,8
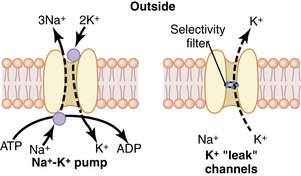
(From Hall JE: Guyton and Hall textbook of medical physiology, ed 12, Philadelphia, 2011, Saunders.)
Depolarization results in an intracellular change from negative to positive. This occurs because Na+ specific channels are opened (or activated), allowing Na+ to enter the cell. As Na+ enters the cell, additional Na+ channels are opened, resulting in a positive feedback loop. Potassium-specific channels are opened, allowing K+ to move out of the cell and into the extracellular space. When membrane potential becomes reversed, to approximately +35 millivolts, the movement of Na+ into the cell and K+ out of the cell stops (Na+ channels become inactivated) and the Na+/K+ pump restores the membrane potential back to a resting state (Na+ channels are at a resting state; Fig. 24-3).4,7,8
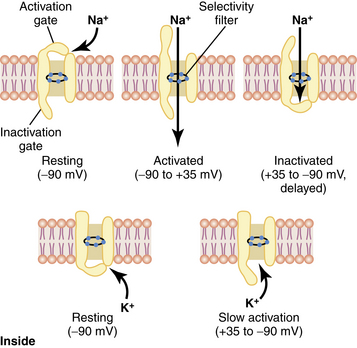
FIG. 24-3 Characteristics of the voltage-gated sodium (top) and potassium (bottom) channels.
(From Hall JE: Guyton and Hall textbook of medical physiology, ed 12, Philadelphia, 2011, Saunders.)
An action potential at a singular point will cause additional areas to also depolarize; this is called propagation. Action potentials must reach threshold to continue propagation (all or none response). In unmyelinated nerves, action potentials are propagated along Na+ and K+ gates located in close proximity, and the conduction rate is slow (0.1 to 2.0 m/sec; Fig. 24-4). Myelinated nerves are, in effect, insulated. Ions cannot move through the myelin. Nodes of Ranvier represent an interruption in the myelin. It is here that action potentials can stimulate depolarization and propagation of an impulse. Because the nodes of Ranvier are spaced farther apart, saltatory conduction occurs, resulting in a faster conduction rate (3-120 m/sec; Fig. 24-5).4,7,8
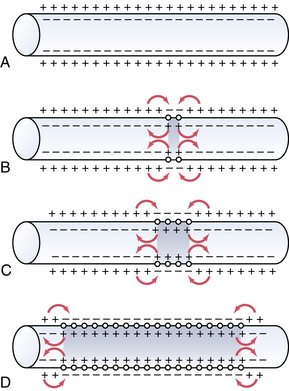
(From Hall JE: Guyton and Hall textbook of medical physiology, ed 12, Philadelphia, 2011, Saunders.)
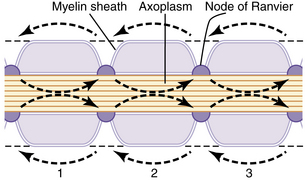
(From Hall JE: Guyton and Hall textbook of medical physiology, ed 12, Philadelphia, 2011, Saunders.)
Local anesthetics work at the level of the Na+ channel to prevent action potentials from being potentiated and not by altering resting or threshold potential. Local anesthetic molecules are stereospecific and bind within the Na+ channel or near its opening. Local anesthetic molecules have a greater affinity for receptors during their open or inactive state. When local anesthetic molecules bind with the Na+ channel, it prevents a conformational change and the influx of Na+, and depolarization slows. Eventually the minimum blocking concentration of the local anesthetic prevents an action potential from being achieved, and electrical conduction is blocked (Fig. 24-6).4,7,9
Nerve fiber type and local anesthetic effects
Peripheral nerves are divided into three classifications based on diameter, myelination, and conduction velocity. A and B peripheral nerves are myelinated, whereas C fibers are not. A fibers have the fastest conduction velocities, followed by B and C fibers. A fibers are further divided into alpha (α), beta (β), gamma (γ), and delta (δ) fibers. A-α fibers have the fastest conduction velocities and are motor neurons (efferent). A-β fibers are slower than A-α fibers but similar to A-γ fibers in conduction velocity, and are sensory (afferent) neurons that detect touch, pressure, and proprioception. A-γ fibers have a similar conduction speed to A-β fibers and are motor neurons (efferent) to muscle spindles and responsible for reflexes. A-δ fibers have the slowest conduction velocity of the A fibers, are sensory (efferent) for sharp pain (also known as fast pain such as an incision or pin prick) and temperature. B fibers have a slower conduction velocity than the A fibers and are preganglionic sympathetic neurons (efferent). C fibers are unmyelinated, have the slowest conduction velocity, and are postganglionic sympathetic neurons (efferent). C fibers transmit slow pain (generalized ache or burning sensation) and temperature (Table 24-1).3,4,7,9
Table 24-1 Classification of Nerve Fibers
FIBER TYPE | MYELIN | FUNCTION |
---|---|---|
A-α | +++ | Motor (efferent: to skeletal muscle) |
A-β | ++ | Touch, pressure, proprioception (afferent: from skin) |
A-γ | ++ | Motor (efferent: to muscle spindles) |
A-δ | ++ | Pain (sharp, fast) and temperature (efferent: from skin) |
B | + | Preganglionic sympathetic (efferent: to vascular smooth muscle) |
C | None | Pain (dull, slow) and temperature (afferent from skin); postganglionic sympathetic (efferent: to vascular smooth muscle) |
From Schick L, Windle PE, editors: Perianesthesia nursing core curriculum: preprocedure, phase I and phase II PACU nursing, ed 2, St. Louis, 2010, Saunders.
Classification of peripheral nerves is important in determining the sequence of local anesthetic blockade. B fibers are the most sensitive. Dilation of cutaneous blood vessels is often the first sign of local anesthetic onset. C fibers and A-δ are next in sensitivity, resulting in the inability to feel cool sensations such as an alcohol wipe. Next in sensitivity are the A-γ, A-β, and A-α fibers, which result in the loss of sensation, pressure, proprioception, and finally motor paralysis. Local anesthetic concentration must be adequate to block nerve fibers. It takes twice the concentration of local anesthetic to block motor fibers as it does to block sensory and sympathetic fibers require the least. The order of blockade onset is B fibers > C fibers = A-δ fibers > A-γ fibers > A-β fibers > A-α fibers. Recovery from local anesthetic is the opposite (Box 24-1).3,4,7,9
Local anesthetic pharmacology
Chemistry and stereoisomerism
A local anesthetic molecule contains a lipophilic group, an intermediate bond, and a hydrophilic group. The lipophilic (attracted to fat) portion of the molecule is aromatic, usually an unsaturated benzene ring. Classification is dependent on the intermediate bond, which is either an ester (–CO–) or an amide (–HNC–). The hydrophilic (attracted to water) group is generally a tertiary amine and proton acceptor (Fig. 24-7).4,10,11 The lipophilic and hydrophilic portions of the molecular structure are crucial to its ability to cross tissue barriers and reach their site of action. The intermediate bond determines the type of local anesthetic, affecting metabolism and potential for allergic reactions.4 Commonly administered amides and esters are noted in Box 24-2.
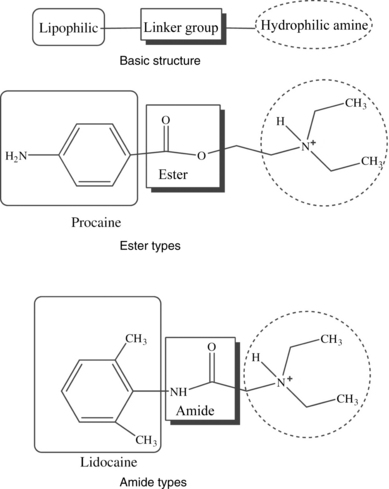
FIG. 24-7 Chemical structure of local anesthetics.
(From Friel CJ, et al: Local anesthetic use in perioperative areas, Perioperative Nurs Clin 5:203–214, 2010.)
BOX 24-2 Local Anesthetics
Amides | Esters |
---|---|
Bupivacaine | Benzocaine |
Etidocaine | Chloroprocaine |
Levobupivacaine | Cocaine |
Lidocaine | Procaine |
Mepivacaine | Tetracaine |
Prilocaine | |
Ropivacaine |
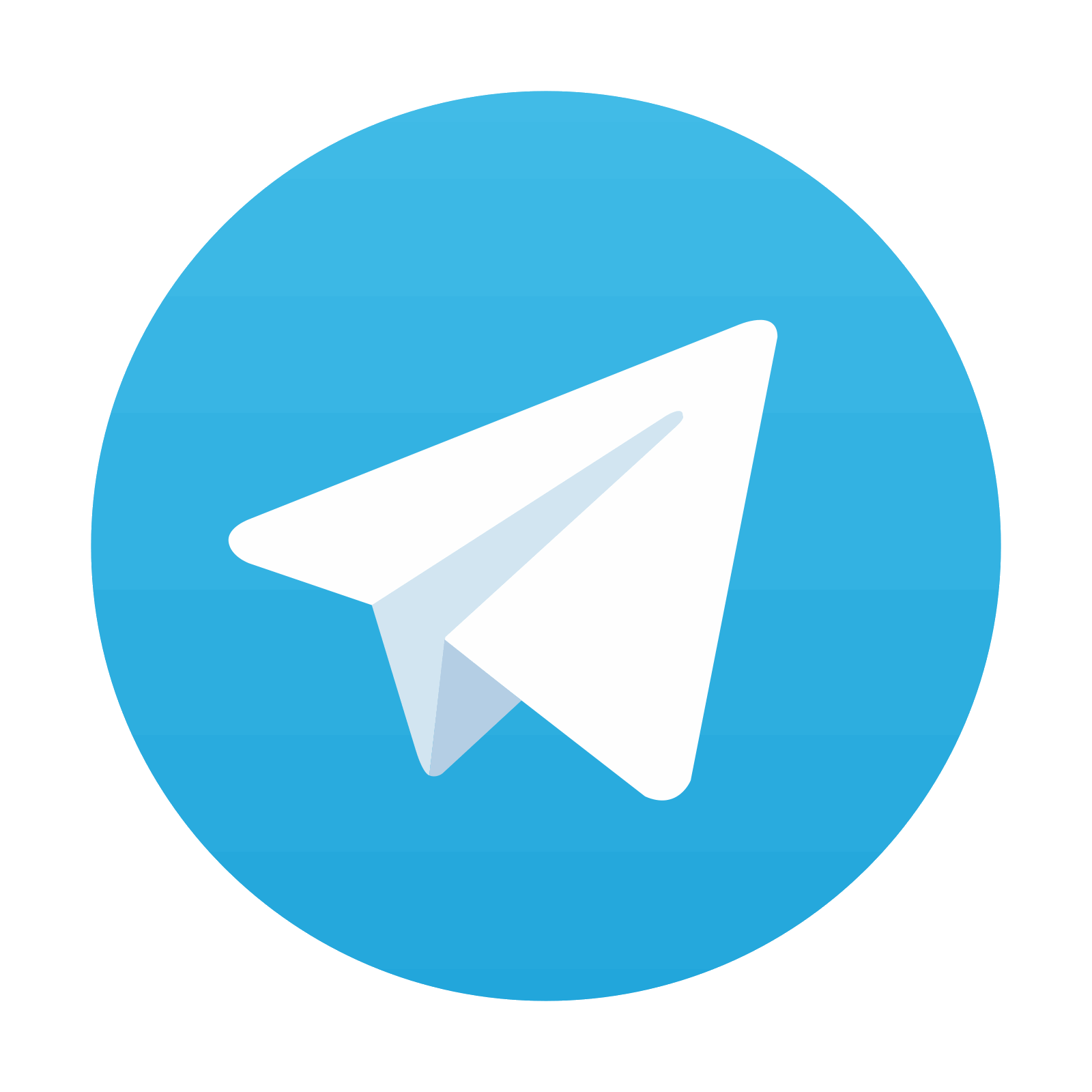
Stay updated, free articles. Join our Telegram channel
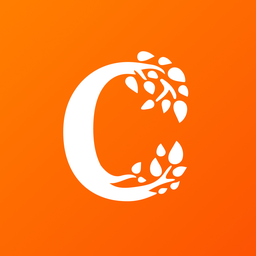
Full access? Get Clinical Tree
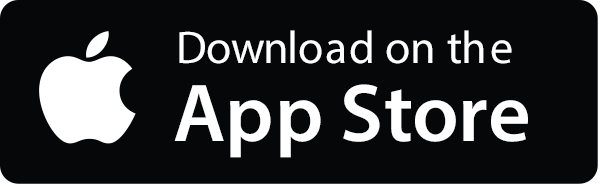
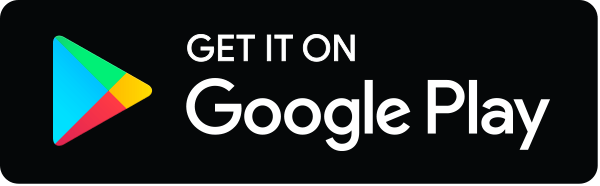