12 Fluid, Electrolyte, and Endocrine Problems
Pearls
• The term osmolality refers to the concentration of solute (electrolytes and proteins) per liter of fluid. Serum osmolality reflects extracellular fluid osmolality. It can be estimated with the following formula:
• Acute changes in serum sodium and osmolality can cause acute water shifts between the intracellular and extracellular spaces. An acute fall in serum sodium concentration and osmolality and the resulting intracellular water shift can cause cerebral edema. If neurologic symptoms develop, urgent treatment is needed. In general, significant water shifts into and out of the vascular space are poorly tolerated.
• Critical care practitioners use the child’s estimated maintenance fluid requirement as a baseline and individualize administered fluid and electrolytes to meet patient needs.
• With the development of acidosis or alkalosis, the serum potassium concentration will change in a direction opposite the change in serum pH, in response to reciprocal potassium and hydrogen ion shifts into and out of the cell.
• If the level of consciousness of the child with diabetic ketoacidosis (DKA) deteriorates during treatment, cerebral edema may be present and urgent intervention is needed. If clinical signs of cerebral edema develop, immediate treatment with intravenous (IV) mannitol or hypertonic saline is needed. If the child’s ability to protect the airway or spontaneous ventilation deteriorates, intubation and mechanical ventilation are indicated.
Anatomy and physiology
Fluid Compartments
Water accounts for 65% to 80% of total body weight. The total body water (TBW) volume and distribution are influenced by factors such as age, gender, adipose content, and skeletal muscle mass (Table 12-1). TBW is divided into two compartments (see Evolve Table 12-1 and Evolve Fig. 12-1 in the Chapter 12 Supplement on the Evolve Website for more information): intracellular fluid (ICF) and extracellular fluid (ECF) compartments (Fig. 12-1).
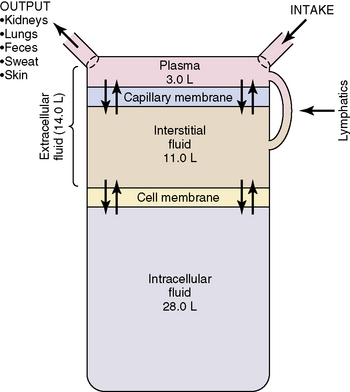
Fig. 12-1 Body fluid compartments showing values for an average 70-kg person.
(From Guyton AC, Hall JE, editors: Textbook of medical physiology, ed 11. Philadelphia, 2006, WB Saunders, p. 292, Fig. 29-1.)
Developmental Considerations
Energy requirements (per kilogram body weight) and metabolic rate are higher in infancy and childhood (for further information, see Chapter 14). The ratio of BSA to volume is significantly higher in infants and children than in adults. As a result, pediatric evaporative fluid losses and fluid requirements per kilogram body weight are higher than those of adults.
Insensible water loss (IWL) is fluid lost through the skin, via evaporation and sweat, and through the respiratory tract. Normal IWL is approximately 300 to 400 mL/m2 BSA per day (for more detailed information, see Evolve Table 12-2 in the Chapter 12 Supplement on the Evolve Website). Fever increases IWL by approximately 0.42 mL/kg per hour for each degree Celsius increase above 37° C. Increased IWL can occur with increased air movement across the skin, and with tachypnea, unless inspired air is humidified. IWL decreases when ambient and inspired air are humidified.
Table 12-2 Formulas for Estimating Daily Maintenance Fluid and Electrolyte Requirements for Children
Daily Requirements | Hourly Requirements | |
Fluid Requirements Estimated from Weight* | ||
Newborn (up to 72 hr after birth) | 60-100 mL/kg (newborns are born with excess body water) | – |
Up to 10 kg | 100 mL/kg (can increase up to 150 mL/kg to provide caloric requirements if renal and cardiac function are adequate) | 4 mL/kg |
11-20 kg | 1000 mL for the first 10 kg + 50 mL/kg for each kg over 10 kg | 40 mL for first 10 kg + 2 mL/kg for each kg over 10 kg |
21-30 kg | 1500 mL for the first 20 kg + 25 mL/kg for each kg over 20 kg | 60 mL for first 20 kg + 1 mL/kg for each kg over 20 kg |
Fluid Requirements Estimated from Body Surface Area (BSA) | ||
Maintenance | 1500 mL/m2 BSA | – |
Insensible losses | 300-400 mL/m2 BSA | – |
Electrolytes | ||
Sodium (Na) | 2-4 mEq/kg | – |
Potassium (K) | 1-2 mEq/kg | – |
Chloride (Cl) | 2-3 mEq/kg | – |
Calcium (Ca) | 0.5-3 mEq/kg | – |
Phosphorous (Phos) | 0.5-2 mmol/kg | – |
Magnesium (Mg) | 0.4-0.9 mEq/kg | – |
* The “maintenance” fluids calculated by these formulas must only be used as a starting point to determine the fluid requirements of an individual patient. If intravascular volume is adequate, children with cardiac, pulmonary, or renal failure or increased intracranial pressure should generally receive less than these calculated “maintenance” fluids. The formula utilizing body weight generally results in a generous “maintenance” fluid total.
Fluid and electrolyte requirements will vary with age and clinical condition. Normal baseline fluid and electrolyte requirements are listed in Table 12-2. Critical care practitioners use estimated maintenance fluid requirements as a baseline and individualize administered fluid and electrolytes to meet patient needs.
Fluid, Electrolyte, and Glucose Balance
Role of Osmolality
The term osmolality refers to the concentration of solute (electrolytes and proteins) per liter of fluid. Serum osmolality reflects ECF osmolality. It can be estimated with the following formula*:
Changes in the osmolality of one body fluid compartment will affect all other compartments. Water shifts between the ICF and the ECF compartments in response to changes in the osmolality of either compartment, moving from the compartment of lower osmolality to the compartment of higher osmolality until osmolality equilibrates. When the osmolality of the extracellular compartment (including the vascular space) decreases, water will shift from the extracellular compartment into cells (Fig. 12-2). Conversely, when the osmolality of the extracellular compartment (including the vascular space) increases, water will shift from the intracellular to the extracellular compartment (including into the vascular space). The volume and acuity of the water shift, as well as likely clinical significance, is determined by the magnitude and acuity of the osmolality gradient between compartments. Significant water shifts can cause neurologic complications.
Renal Influences
The kidneys help maintain fluid balance through filtration and selective reabsorption. Changes in the glomerular filtration rate (GFR) alter the amount of water and sodium excreted or reabsorbed by the kidneys. Expansion of intravascular volume normally increases the GFR, increasing sodium and water excretion. When intravascular volume is depleted, the GFR falls and sodium and water excretion decrease (i.e., more sodium and water are reabsorbed into plasma from the renal filtrate). As noted previously, the kidney is less able to concentrate urine during the first months of life. (See Chapter 13 for more detailed information.)
Endocrine Influences
Several hormones contribute to regulation of fluid and electrolyte balance.
Antidiuretic Hormone (ADH, Vasopressin)
Negative feedback mechanisms normally regulate ADH secretion to maintain a serum osmolality of 275 to 295 mOsm/L. Osmolality receptors located in the brain are stimulated by a rise in serum osmolality (e.g., above 285 mOsm/L or a rise of at least 2%). Volume-sensitive receptors (located in the left atrium and thoracic vessels) and baroreceptors (stretch receptors located in the ascending aorta, pulmonary arteries, and carotid sinus) are stimulated by volume depletion and hypotension. Additional causes of ADH secretion include stress, trauma and severe pain (through activation of cholinergic neurotransmitters in the hypothalamus), angiotensin II, and some medications.3 A normal or low serum osmolality, hypertension, and an increase in left atrial stretch should inhibit ADH secretion.
Although endogenous ADH does not affect vascular tone, exogenous (administered) vasopressin can cause vasoconstriction and increase blood pressure. For further information regarding use vasopressin, see Chapters 6 and 14.
Serum Glucose in Critically Ill or Injured Children
Although a serum glucose concentration of 60 to 180 mg/dL is normally maintained over a wide range of conditions, critically ill or injured children often develop hypoglycemia or hyperglycemia. Infants have high glucose needs and low glycogen stores, so they can rapidly become hypoglycemic during critical illness or injury.24 Providers should monitor serum glucose concentration with point-of-care testing, if possible, and treat hypoglycemia as needed. Treatment of hypoglycemia should avoid frequent, intermittent bolus administration of large quantities of glucose; provision of a continuous source of glucose is preferable.
Hyperglycemia can result from steroid administration, stress response, relative hypoinsulinemia, or insulin resistance and has been associated with increased mortality in critically ill children in some studies. A prospective, randomized study of tight control of serum glucose concentration in critically ill children (targeted to age-adjusted normal fasting glucose concentration) reduced critical care unit mortality,37 but was associated with episodes of hypoglycemia. In general, an insulin infusion (0.5-1 unit regular insulin/kg per hour) is often titrated during the first 18 to 24 hours of critical care therapy to maintain the serum glucose concentration less than 150 mg/dL (range will vary; use your unit protocol). Careful monitoring is required to avoid and treat episodes of hypoglycemia. The ultimate value versus risk of this approach is still under investigation.
Electrolyte homeostasis and common imbalances
Table 12-3 presents a summary of electrolyte imbalances and associated clinical manifestations in critically ill infants and children. In the following sections, approximate ranges for normal and abnormal serum electrolyte concentrations are listed, but providers should use normal ranges referenced by the clinical laboratory in their practice settings.
Sodium Imbalance: Hyponatremia
Etiology
Hyponatremia is a low serum sodium concentration, typically less than 130 to 135 mEq/L. It often develops as a complication of disease or therapy. The critically ill infant or child can develop hyponatremia from excessive water intake relative to sodium, excess water retention, increased sodium loss, or a combination of these factors.10
Hyponatremia can occur in conjunction with hypervolemia, euvolemia, or hypovolemia. Hypervolemic hyponatremia is associated with water intoxication, nephrotic syndrome, cardiac failure, renal failure, and the syndrome of inappropriate antidiuretic hormone (SIADH). Hypovolemic hyponatremia can occur with renal losses (e.g., osmotic diuresis, renal tubular acidosis) or extrarenal losses (e.g., diarrhea, vomiting, burns).10 Other potential causes include adrenal insufficiency, excessive use of diuretics, and cerebral salt-wasting syndrome.
Pathophysiology and Clinical Signs and Symptoms
The volume of water shift and the severity of clinical manifestations with hyponatremia are directly related to the acuity and the magnitude of the fall in serum sodium and osmolality. Infants and children who develop hyponatremia that gradually worsens over several days or weeks (e.g., with adrenocortical insufficiency) typically have milder clinical manifestations and may be asymptomatic until the serum sodium is very low.26
Acute hyponatremia that develops within hours or days (i.e., <48 hours) is more likely to produce cerebral edema.26 Seizures and coma are associated with a serum sodium concentration less than 120 mEq/L.
Management
Once the child’s neurologic status is stable and perfusion is adequate, plans are made to replace the sodium deficit. The following formula36 is used to calculate the sodium deficit:
During treatment of hyponatremia, providers must closely monitor the serum sodium concentration and the rate of rise in the concentration. If the serum sodium and osmolality are raised too rapidly, the resulting water shifts from the cellular to the extracellular (including intravascular) compartments can produce neurologic complications including intracranial bleeding (Box 12-1). In general, the serum sodium should be raised no faster than approximately 0.5-1.0 mEq/L per hour.35 Additional important assessments include strict monitoring of intake and output, urine specific gravity, serum electrolytes, serum osmolality, and daily weights.
Rapid correction of hyponatremia has been shown to result in cerebral dysfunction, linked with damage to the myelin sheath of neurons. This myelinolysis is called central pontine myelinolysis if it occurs in the pons (brainstem) and osmotic demyelinization syndrome if it occurs elsewhere in the brain. Signs of brain dysfunction can include decreased level of consciousness, lack of coordination, paralysis, and dysphagia. Because there is no known treatment for such cerebral dysfunction, and it can cause permanent disability, prevention is critical. In general, unless neurologic symptoms indicate the need for more aggressive treatment, providers should aim to correct hyponatremia or hypernatremia no faster than approximately 0.5 mEq/L per hour (or 10-12 mEq/L per day). For more information, consult the National Institutes of Health Web site: http://www.ninds.nih.gov/disorders/central_pontine/central_pontine_myelinolysis.htm.
Sodium Imbalances: Hypernatremia
Pathophysiology and Clinical Signs and Symptoms
Hypernatremia typically increases the serum osmolality. The rise in osmolality stimulates the posterior pituitary to release ADH, which increases renal water reabsorption until the osmolality returns to normal. The increased osmolality associated with hypernatremia also leads to a shift in water from the intracellular to the extracellular compartment, including into the vascular space. This water movement from the cells can cause cellular dehydration and central nervous system dysfunction. Complications including subdural, subarachnoid, and intracerebral bleeding and sinus vein thrombosis can develop with acute and severe increases in serum sodium concentration and the resulting water shift (see Box 12-1). Permanent central nervous system dysfunction can result when the serum sodium concentration is extremely high (e.g., >165-170 mEq/L).12 When serum osmolality is chronically elevated, brain cells will generate idiogenic osmoles to maintain cell volume (Box 12-2).
Management
If the patient with hypernatremia has signs of inadequate tissue perfusion (i.e., shock), administer isotonic crystalloid by bolus (20 mL/kg) until perfusion is adequate. Avoid excessive bolus fluid administration (i.e., beyond that needed to treat shock), because it can contribute to a rapid fall in serum sodium and osmolality resulting in cerebral edema and other neurologic complications (see Box 12-2).
Potassium Homeostasis
The normal serum potassium (K+) concentration is 3.0 to 5.0 mEq/L. The intracellular potassium concentration is much higher, approximately 150 mEq/L.28 Small alterations in serum potassium concentration can significantly affect the transmembrane gradient and therefore neuromuscular and cardiac function. The serum K+ concentration is affected by potassium intake and excretion, renal regulation, and serum pH. Because the intravascular (serum) potassium concentration represents only a small proportion of the total body potassium, accurate interpretation of the child’s potassium balance requires consideration of the child’s clinical status and acid-base balance.
Potassium ions normally shift between the intra- and extracellular compartments with changes in the serum pH (see Evolve Fig. 12-2 in the Chapter 12 Supplement on the Evolve Website). When the serum hydrogen ion concentration increases (i.e., with acidosis or a fall in pH), hydrogen moves from the extracellular to the intracellular compartment, where it is buffered. To maintain a balance of cation movement across cell membranes, the intracellular movement of hydrogen ions is associated with an extracellular shift of potassium. Thus, acidosis or a fall in pH (e.g., with correction of alkalosis) is associated with a rise in serum potassium concentration. In contrast, alkalosis or a rise in serum pH (e.g., with treatment of acidosis) is associated with a fall in serum potassium, because hydrogen ion shifts out of cells and is replaced by potassium.
The kidneys play a critical role in potassium homeostasis. Renal failure limits the kidney’s ability to excrete potassium and may result in hyperkalemia (see Chapter 13).
Potassium Imbalances: Hypokalemia
Etiology
Causes of hypokalemia (serum K+ concentration <2.5 to 3.0 mEq/L) can be classified into three general categories: inadequate potassium intake (rare, but may be iatrogenic), shifts of potassium from the extracellular to the intracellular compartment (e.g., with alkalosis or a rise in serum pH), or excessive losses of potassium (see Evolve Fig. 12-3 in the Chapter 12 Supplement on the Evolve Website for more information). Critically ill patients often develop true potassium deficit following the use of diuretics, especially loop and thiazide diuretics. Some antimicrobial agents (e.g., amphotericin B or carbenicillin) can increase renal potassium losses. Hypokalemia also is associated with severe hypochloremia and the potassium-wasting Bartter’s syndrome.
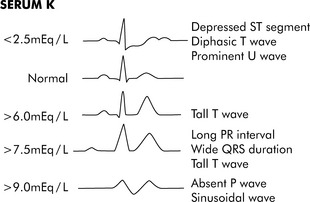
Fig. 12-3 Electrocardiogram changes with hypokalemia and hyperkalemia.
(From Park MK, Guntheroth WG: How to read pediatric ECGs, ed 3, St. Louis, 1992, Mosby, p. 108.)
Pathophysiology and Clinical Signs and Symptoms
Hypokalemia can cause hyperpolarization of nerve and muscle cells, leading to muscle weakness, slowed nerve impulse conduction, and decreased muscle contraction. Hypokalemia can cause characteristic electrocardiogram (ECG) changes, including development of a U-wave (Fig. 12-3).
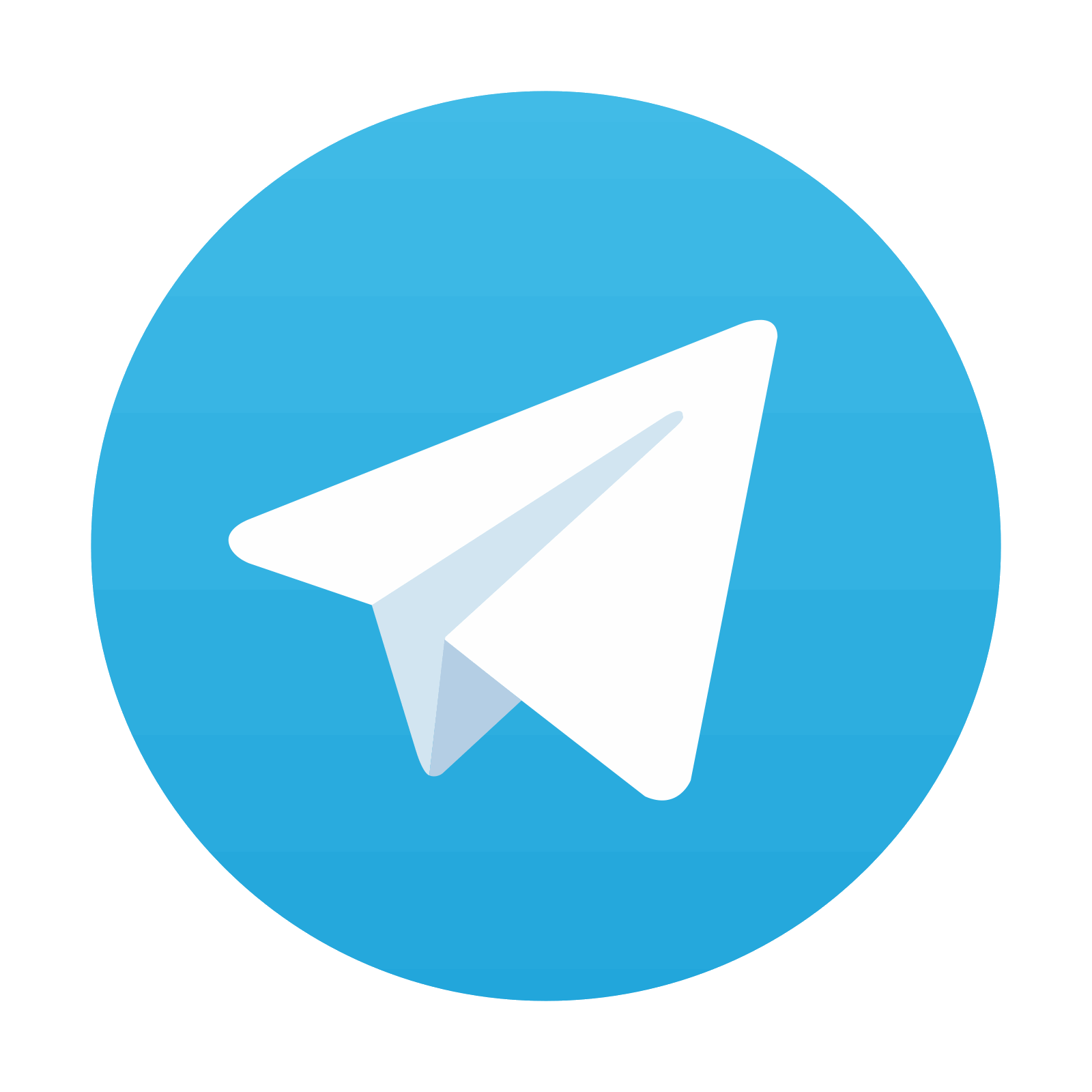
Stay updated, free articles. Join our Telegram channel
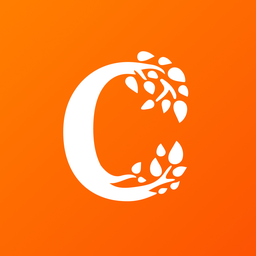
Full access? Get Clinical Tree
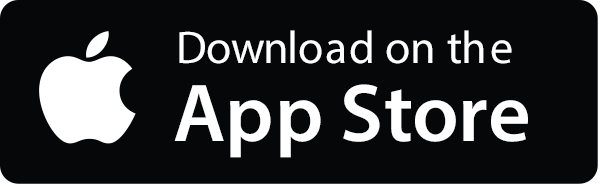
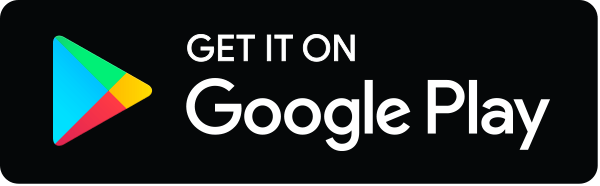