On the concave surface of the kidney lies the hilus, from which the ureter and the main blood vessels and nerves access the kidney. The cut surface of the kidney reveals two distinct regions: a dark outer region, the cortex and a pale inner region called the medulla. The outer cortex is covered by a fibrous capsule and the whole kidney is surrounded by a pad of fat that offers some protection against injury. Broadly speaking, the cortex contains the filtering and reabsorptive components of the nephrons, whilst the medulla contains the concentrating and diluting components of the nephrons and a system of collection ducts. These ducts funnel the urine into the pelvis at the heart of the medulla, from where it moves down the ureter into the bladder (Figure 2.2).
The nephron
The nephron is the functional unit of the kidney and each kidney contains approximately 1 million nephrons (Figure 2.3). The unique structure of the nephron is critically related to its complex functions and contains five components, each performing a distinct process:
Figure 2.3 The parts of a nephron, collecting duct, and associated blood vessels.
Source: Tortora and Derrickson (2007), Introduction to the Human Body, The Essentials of Anatomy and Physiology, 7th edition. This material is reproduced with permission of John Wiley & Sons, Inc.
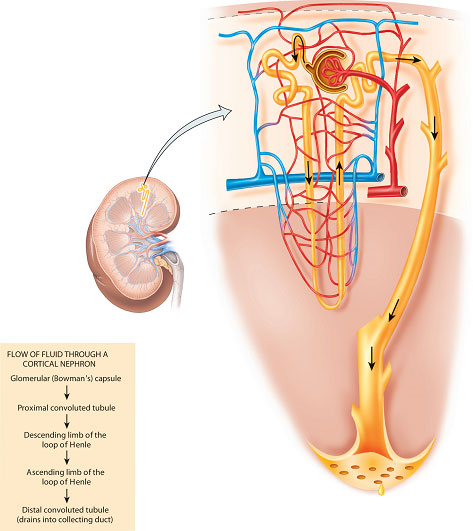
- the Bowman’s capsule – forming a blind-ending capsule around a knot of capillaries called the glomerulus (the site of filtration);
- the proximal convoluted tubule (the site of bulk-phase reabsorption and some secretion);
- the loop of Henle (where the concentration and dilution of urine mainly occur);
- the distal convoluted tubule (the site of ‘fine-tuning’ reabsorption and more secretion);
- the collecting duct (also important for the concentration of urine and for carrying urine into the renal pelvis).
These processes do not occur in isolation but are interdependent and intimately related to each other by the shape of the nephron. The importance of this fact will become clear when the renal processes are discussed in more detail.
There are broadly two types of nephron found in the kidney (Figure 2.4). Approximately 85% of nephrons are cortical nephrons, which have short loops of Henle that are contained in the cortex of the kidney. The other 15% of nephrons are called juxtamedullary nephrons and they have long loops of Henle, which extend deep into the medulla of the kidney. It is the long loops of Henle that enable concentration of urine; animals adapted to arid environments have long loops of Henle compared with those that have a lesser requirement to conserve water. It is the loops of Henle, together with the collecting ducts which also pass through the medulla, that give the pyramids of the medulla a striated appearance.
The main functions of the kidney are to rid the body of the end-product of metabolism and to regulate the electrolytes found in the body fluids. A more detailed list of the functions of the kidney can be found in Box 2.1. Before looking at how the kidney achieves its functions, there follows a discussion on the impressive versatility of urine.
- Excretion of metabolic waste products, e.g. urea and creatinine.
- body water volume;
- body fluid osmolality;
- electrolyte balance;
- acid–base balance;
- blood pressure.
- Activation of vitamin D.
- Production of renin.
- Production of erythropoietin.
The versatility of urine
When the immense variability in the qualities of urine that can be produced in order to control our internal environment is considered, one cannot fail to be impressed by the complexities of the workings of the kidney. Broadly speaking, there are three parameters that can be varied in order to maintain the constancy of our bodily fluids: urinary volume, urinary concentration and urinary content.
Urinary volume
In a healthy person, the volume of urine produced per day can vary from as little as 300 ml, if no water is ingested or there is excessive water loss from the body (as in diarrhoea), up to a maximum of 23 L in cases of excessive fluid ingestion. In health, urine output should not drop below 300 ml/day because this is the absolute minimum water volume required to excrete the daily load of toxic waste products. If the amount of waste products to be removed by the kidney rises, then the minimum urine volume must also rise. However, the average urine output per day is approximately 1500 ml. The kidneys’ ability to vary the volume of daily urine output over such a wide range is essential if we are to maintain a constant body fluid volume in the face of adverse factors such as excessive heat, which causes sweating; colonic infections causing diarrhoea; or excessive thirst and water ingestion, as seen in the condition psychogenic polydipsia (Table 2.1).
Table 2.1 Normal fluid inputs and outputs.
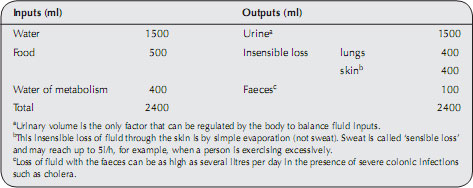
Urinary concentration
Though the volume of urine can vary over a wide range, the amount of solutes to be excreted by the kidney each day is much less variable. Thus, in order to excrete a fairly fixed volume of solutes each day in a very variable volume of water, the kidney must have the ability to concentrate or dilute the urine. On a hot summer’s day when very little fluid has been drunk, urine is dark in colour and of low volume, whereas if liberal amounts of beer have been consumed at a party, large volumes of watery urine are passed all evening. In fact this diuretic effect of beer is not wholly due to the volume imbibed, but also to the fact that the alcohol present in the beer suppresses the secretion (from the posterior pituitary gland) of antidiuretic hormone (ADH, also known as vasopressin), a hormone that would normally prevent diuresis. The hangover suffered the next day is therefore caused by dehydration.
The ability of the kidneys to excrete all the body’s excess solutes in varying amounts of water by concentrating or diluting the urine is essential for maintaining a constant body osmolality (Box 2.2). The mechanism in the kidney that controls the concentration or dilution of urine is often affected early on in renal disease, making it difficult for the individual to control both body fluid volume and osmolality in response to changes in fluid inputs and outputs. This can result in the individual tipping back and forth from states of dehydration to fluid overload.
- Water can move between the different body fluid compartments across semipermeable membranes by the process of osmosis.
- The more concentrated a solution, the more water will be drawn into this solution.
- Any solute that can cause the movement of water across a semipermeable membrane is said to be ‘osmotically active’.
- The osmotic activity of substances in solution is dependent on the number of dissolved particles in the solution and not on their size or charge.
- Sodium chloride (NaCl) dissociates in solution into Na+ and Cl– ions, so the number of osmotically active particles is almost double the number of NaCl molecules (but not exactly double because the dissociation is not complete; the solution consists of NaCl, Na+ and Cl– particles).
- Osmotically active particles in solution are measured by the unit called the osmole or milliosmole (mosmol).
- Osmolality is measured in mosmol/kg water and is a measure of the potential osmotic activity of dissolved solutes in solution.
- Normal body fluid osmolality is 285 mosmol/kg water.
- The concentration (rather than the osmotic activity) of individual electrolytes is measured in mmol (millimoles) or μmol (micromoles) rather than mosmol, e.g. normal fasting blood glucose range = 3.6–5.8 mmol/l
Urinary content
The range of substances that can be constituents of urine is varied and includes:
- ions: sodium, potassium, calcium, magnesium, chloride, bicarbonate, phosphate and ammonium;
- metabolic waste: urea, creatinine and uric acid;
- drug metabolites: most metabolites of pharmacological agents are eventually excreted from the body through the kidneys; many are detoxified in the liver first;
- other products of normal metabolism: metabolites of hormones can be detected in the urine by appropriate assays and may be a diagnostic aid, for example, the appearance of human chorionic gonadotrophin (hCG) in the urine in the early stages of pregnancy forms the basis of the pregnancy test.
Normal urine is clear in appearance, though it may vary in colour from pale to dark amber, depending on its concentration. It has no unpleasant odour, though urine that has been standing a long time may develop a strong ammonia smell. Finally, normal urine has a pH that is slightly acidic – around pH 6 – though urine can have a pH in the range 4.0–8.0 in cases of severe acidosis or alkalosis respectively.
Basic Renal Processes
Glomerular filtration
This is a process of filtration of plasma across the glomerular basement membrane from the glomerulus into the Bowman’s capsule (Figure 2.5). The glomerular filtration surface is a unique structure composed of three layers:
Figure 2.5 Structure of the glomerulus and Bowman’s capsule.
Source: Tortora and Nielsen (2009) Principles of Human Anatomy, 11th edition. This material is reproduced with permission of John Wiley & Sons, Inc.
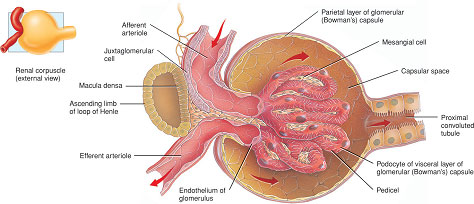
- the endothelial lining of the glomerular capillaries;
- the basement membrane;
- the epithelial cells of the Bowman’s capsule, or podocytes. Filtration occurs between the slits formed by the finger-like processes of these cells (called pedicels) which surround the glomerular capillaries (Figure 2.5).
These three layers are fused together and act as a barrier to the filtration of large-molecular-weight molecules such as proteins.
Blood enters the glomerulus from a series of branches of the renal artery, ending in the afferent arteriole. Blood then leaves the glomerulus through the efferent arteriole rather than a vein. In the majority of nephrons (those situated in the cortex), the efferent arteriole from the glomerulus divides up into capillaries, which cover the surfaces of the convoluted tubules. The capillaries finally empty into the venous system. In the deeper juxtamedullary nephrons the efferent arteriole from the glomerulus divides to form loops which lie parallel to the loops of Henle and so run down into the medulla. These vessels are called the vasa recta and are concerned with the process of concentration of the urine (Figure 2.6). Blood then leaves the kidney through a series of larger converging veins, ending in the renal vein which returns the blood to the vena cava.
Figure 2.6 Blood supply of the right kidney.
Source: Tortora and Derrickson (2007), Introduction to the Human Body, The Essentials of Anatomy and Physiology, 7th edition. This material is reproduced with permission of John Wiley & Sons, Inc.
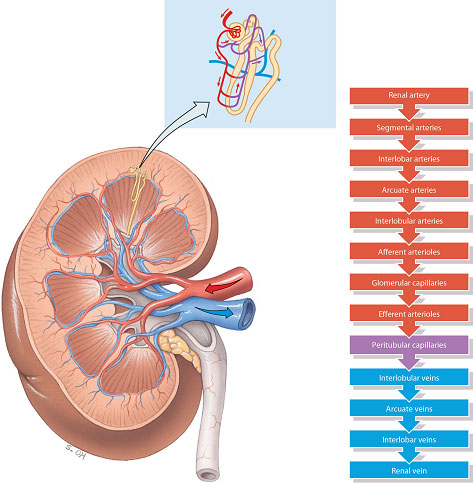
In effect, the nephrons contain two capillary beds, one within the glomerulus and a second which surrounds the convoluted tubules of the nephrons. This unusual arrangement of two capillary beds in tandem enables a pressure gradient to exist within the nephron, with high pressure in the glomerulus, favouring filtration, and a relatively low pressure in the peritubular capillaries, favouring reabsorption.
The formation of a filtrate from plasma flowing through the glomerulus occurs due to Starling’s forces. These are the same forces that cause the filtration and reabsorption of tissue fluid in other capillary beds in the body, but with some important adaptations. In the glomerulus, filtration depends on three main pressures. One type of pressure promotes filtration, and the other pressures oppose filtration. Fluid is forced across the glomerular basement membrane because of the high hydrostatic pressure of blood flowing through the afferent arteriole. This pressure is greater than the sum of the blood colloid osmotic pressure (BCOP) and the pressure in the Bowman’s capsule (capsular hydrostatic pressure) opposing it (Figure 2.7). In an ordinary capillary bed, this filtrate would be almost entirely reabsorbed back into the capillary at the venule end because the hydrostatic pressure would have fallen to below the colloid osmotic pressure of the capillary, which would then pull the fluid back in by osmosis. However, in the glomerulus, because blood leaves via another arteriole (the efferent arteriole) rather than a venule, a high hydrostatic pressure is maintained and the sum of the blood colloid osmotic pressure and capsular hydrostatic pressure is insufficient to move fluid back into the capillary. This situation is obviously desirable so that large amounts of filtrate can be made. All of the filtrate formed in the glomerulus passes into the Bowman’s capsule and is often referred to as tubular urine.
Amount and composition of glomerular filtrate
The amount of glomerular filtrate formed per minute is referred to as the glomerular filtration rate (GFR), and in the average healthy person is approximately 125 ml/min. This volume is the sum amount from all 2 million nephrons in the kidneys and thus the amount from each nephron is relatively small. A quick calculation shows that at a GFR of 125 ml/min, the total amount of filtrate formed per day is 180 L – approximately 60 times the circulating plasma volume. Obviously, the majority of this must be reabsorbed to prevent severe dehydration.
The ‘average’ GFR quoted is generally only average for a young adult. Children have a lower GFR than this because their nephrons are still increasing in size (all nephrons are present at birth). After the age of about 30 years the number of functioning nephrons starts to decrease because of the ageing process, thus the GFR decreases proportionately. However this only becomes significant to health when the GFR falls to around 5 ml/min – a situation that only occurs in a diseased kidney. An estimated GFR (eGFR) can be calculated clinically by measuring serum creatinine and applying a formula. The principles of the eGFR test are described more fully in Chapters 6 and 7.
The composition of the initial glomerular filtrate is that of a plasma ultrafiltration; that is, without proteins. The main determinant of what can pass through the glomerular basement membrane is molecular size, although the molecular shape and charge are also important. The passage of strongly negatively charged molecules such as albumin tends to be retarded because of the presence of fixed negative charges in the basement membrane which repel their movement. Albumin, a small protein, has a molecular weight of 69 000, a weight just below the cut-off point for filtration. It can therefore cross the filter, but does so only in minute quantities since it is also hindered by its negative charge. Similarly, haemoglobin, with a molecular weight of 68 000, is small enough to be filtered, whereas intact erythrocytes are too large to cross into the Bowman’s capsule. The appearance of haemoglobin in urine therefore indicates haemolysis, with the release of the protein into the blood. It is because of this free permeability of small molecules that the composition of the initial glomerular filtrate is the same as that of the plasma, and will include the major ions sodium, potassium, chloride, bicarbonate, calcium and phosphate; glucose, amino acids and the toxic waste products of urea and creatinine. Any albumin filtered is reabsorbed through the proximal tubule into the renal lymph system and returned to the blood stream.
Selective reabsorption
Reabsorption is a process that involves the movement of water and dissolved substances from the tubular fluid back into the blood stream. The term ‘selective’ infers a regulatory function to this process, as indeed not all of the filtered substances are returned to the blood. Any substances not reabsorbed will pass with the urine into the bladder to be excreted from the body. The main sites for reabsorption in the nephron are the proximal and distal convoluted tubules.
Mechanisms of reabsorption
Broadly speaking, there are three mechanisms in the nephron for the reabsorption of water and solutes: osmosis, diffusion and active transport. Osmosis is the movement of water from an area of low concentration to a more concentrated solution across a membrane which allows water molecules through but is selectively permeable to solute molecules (a semipermeable membrane).
Diffusion occurs where a concentrated solution meets one which is less concentrated. The solute will equilibrate throughout the solvent. This may occur across a membrane so long as the membrane is permeable to the solute. Diffusion may also occur through specialised ‘protein carriers’ in the membrane. This is sometimes known as ‘facilitated diffusion’. In these cases, the number of protein carriers in the membrane is limited, meaning that movement of the solute will be limited. This is true for tubular reabsorption of glucose (which is also linked to sodium transport). Once all the carriers are active, reabsorption of glucose has reached its maximum. If the filtered load of glucose is excessive, not all can be reabsorbed and glucose will appear in the urine, as happens in untreated diabetes mellitus.
Active transport is so named because it is an energy-requiring process. The energy source is adenosine triphosphate (ATP). Active transport can move solutes against a concentration gradient. Cells which carry out a lot of active transport will have a lot of mitochondria present (the ‘power-generating’ organelles of cells).
In the proximal tubule cells, where most reabsorption is by active transport, the cells are packed with mitochondria. Proximal tubule cells also have a large brush border on their luminal surface (the surface facing into the tubule) to increase their surface area for reabsorption. In contrast, cells lining the descending loop of Henle are comparatively thin, have no brush border and have relatively few mitochondria. This suggests that these cells are not very metabolically active and are adapted for reabsorption by passive diffusion. The epithelial cells of the distal tubule are similar to those of the proximal tubule but with a less well-defined brush border and fewer mitochondria. This suggests that these cells are capable of active transport of substances but in much lesser quantities than in the proximal tubule. This again illustrates how each segment of the nephron is anatomically adapted to carry out its unique functions. The different cell types lining the tubules are illustrated in Figure 2.8.
Figure 2.8 Histological features of the renal tubule and collecting duct.
Source: Tortora and Nielsen (2009) Principles of Human Anatomy, 11th edition. This material is reproduced with permission of John Wiley & Sons, Inc.
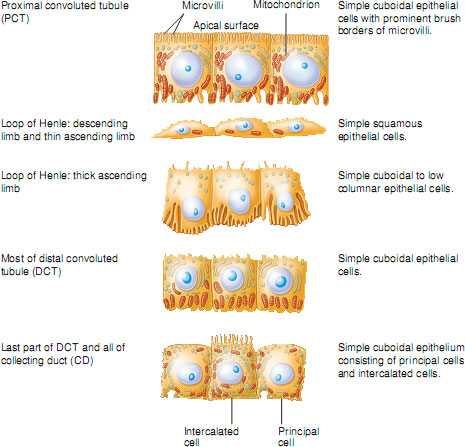
Reabsorption in the proximal convoluted tubule – the site of bulk-phase reabsorption
Approximately 65% of all reabsorption occurs in the proximal tubule (hence the term ‘bulk phase’) and is obligatory rather than regulatory. Most substances here are reabsorbed by active transport mechanisms, including sodium, chloride, potassium, glucose, amino acids, phosphate and bicarbonate. Urea is absorbed by passive diffusion and water is reabsorbed by osmosis. Some substances are reabsorbed almost entirely in the proximal tubule, such as glucose and amino acids, which do not appear in the urine, whereas others have only between 60 and 70% reabsorption, such as sodium, water and potassium. Approximately 50% of urea is reabsorbed here but creatinine is not reabsorbed at all. However, for all substances that are reabsorbed, the proximal tubule is the site where the bulk of this reabsorption occurs.
Reabsorption in the distal convoluted tubule – the site of fine-tuning reabsorption
This is the site where more specific regulation of substances occurs according to the needs of the body. In order for the distal tubule to be aware of precisely what the body’s reabsorptive needs are, there needs to be some method of communication between the cells of the distal tubule and the rest of the body. This communication system is via a range of hormones that form part of a negative-feedback system for the homeostatic control of ions and water. For example, sodium and water reabsorption are under the control of aldosterone (secreted by the adrenal cortex) and ADH secreted by the posterior pituitary gland. Potassium reabsorption is also controlled by aldosterone, whereas calcium and phosphate are controlled by parathyroid hormone (PTH). The precise mechanisms for controlling the electrolytes outlined above will be dealt with in later sections in this chapter.
Secretion
The process of secretion occurs in both the proximal and distal tubules and involves the movement of substances from blood flowing through the peritubular capillaries, through the tubule wall cells, into the tubular fluid. In this respect, secretion is the opposite process to reabsorption. Substances that are secreted into the tubules are excreted in the urine. Though creatinine is freely filtered at the glomerulus, total creatinine excretion is increased by 20% by the process of secretion. Ions that are transported into the tubules by secretion are hydrogen, which is secreted in both the proximal and distal tubules and is important in acid–base control, and potassium, which is secreted in the distal tubule in exchange for sodium reabsorption. The hormone involved is aldosterone.
Excretion of drugs and drug metabolites
Many drugs and their metabolites are finally excreted from the body through the kidneys by the processes of glomerular filtration and secretion. Like other filtered substances, the rate of filtration of drugs will depend on their molecular size and charge: smaller molecules are filtered more rapidly than larger ones. Drugs that bind to plasma proteins are filtered very slowly because of the size of the complex. Some drugs are cleared from the blood purely by glomerular filtration and thus the rate of clearance cannot exceed the GFR of 125 ml/min, whereas other drugs are excreted by a combination of filtration and secretion, such as benzylpenicillin, which achieves a total clearance rate of 480 ml/min (Shitara et al. 2006; Bennett and Brown 2008).
Concentration and dilution of urine
The components of the nephron that are involved in the concentration and dilution of the urine are the loop of Henle and the collecting ducts. The concentration of urine is measured in units of osmolality (mosmol/kg water). The most dilute urine that humans can produce is approximately 60 mosmol/kg water, but this situation occurs in the pathological condition of diabetes insipidus in which the pituitary gland fails to produce ADH. The most concentrated urine that can be produced is approximately 1400 mosmol/kg water, which is greater than four times more concentrated than plasma (plasma osmolality = 285 mosmol/kg water) and requires maximum ADH secretion. The average range of urine osmolality in people with normal kidneys is between 300 and 500 mosmol/kg water.
Countercurrent mechanism of urinary concentration
The countercurrent mechanism is a complex physiological process that will not be discussed in great detail.
In order to concentrate the urine the following factors are required:
- the creation and maintenance of a local environment in the kidney that allows large quantities of water to be reabsorbed by osmosis from the collecting duct back into the blood;
- a mechanism that can influence the opening and closing of water channels in the collecting ducts in order to control the exact amount of water reabsorbed.
Creation of the local environment
This local environment consists of an increasing hyperosmotic medullary interstitium as one moves towards the tip of the loops of Henle. In other words, the tissue spaces between the loops of Henle in the medulla of the kidney must be made hyperosmotic compared to the fluid in the collecting duct. As the collecting ducts pass through the medulla on their way to the renal pelvis, water can be pulled out by osmosis, resulting in less water entering the urine.
This hyperosmotic environment is created by the active and passive transport of ions (mainly sodium and chloride) out of the tubular fluid as it passes through the loop of Henle into the medullary interstitium. The buildup of ions here gradually increases and becomes more concentrated as the loop of Henle descends into the medulla. The tip of the medulla can reach osmolalities of up to 1400 mosmol/kg water. Water does not follow the transport of ions into the medullary interstitium by osmosis because the thick ascending limb of the loop of Henle is impermeable to water. Urea also makes an important contribution to the creation of the hyperosmotic environment. Urea can diffuse passively through the walls of the tubules at most points along the nephron, but urea diffusion is greatly enhanced across the collecting tubule wall in the presence of even small amounts of ADH. Thus large amounts of urea become concentrated in the medullary interstitium.
Maintenance of the local environment: the countercurrent mechanism
It could be thought that as fast as the hyperosmotic environment is created it will be washed out by processes of diffusion and reabsorbed back into blood. However, the environment is maintained because of the unique arrangement of the looped vasa recta capillaries around the loops of Henle (Figure 2.6). The vasa recta are only supplied with 1–2% of the blood entering the kidneys, so there is very little blood flow for ions to be reabsorbed back into. In addition, the vasa recta lie with their ascending and descending limbs in very close proximity. This close proximity allows for rapid exchange of water and solutes between the two limbs. So, as blood flows down the descending limb of the vasa recta, the high concentration of ions in the medullary interstitium enables ions to diffuse into the vasa recta (markedly increasing their concentration), and water to move out by osmosis, but as the blood moves into the ascending limb of the vasa recta, the high concentration of ions in the blood then enables ions to diffuse back out into the medullary interstitium (and water back into the blood), maintaining the hyperosmotic environment.
The regulation of body fluid volume and osmolality
Sodium is the main extracellular cation (positively charged ion) and is intimately related to extracellular volume. An increase in body sodium content could lead to an increase in extracellular fluid volume, leading to hypertension and oedema; and a decrease in body sodium content could lead to a decrease in extracellular fluid volume leading to hypotension and dehydration. Thus, it is vitally important that body sodium content is kept at a constant level if body fluid volume is to remain constant. Fortunately, the healthy kidney conserves sodium very efficiently in shortage, and can excrete 10–150 mmol/day when sodium is in excess.
Sodium levels, and hence fluid volume, are regulated by three hormones:
- ADH;
- aldosterone;
- atrial natriuretic peptide.
Antidiuretic hormone
ADH is secreted from the posterior pituitary gland in response to a rise in plasma osmolality. Osmoreceptors in the hypothalamus detect small changes in the plasma osmolality and send signals to the posterior pituitary to secrete more or less ADH. Since sodium, along with its associated anion (negatively charged ion) chloride (Cl–), contributes approximately 95% of the extracellular fluid osmolality, sodium concentration of extracellular fluid is obviously important in determining ADH secretion.
ADH receptors are found in the collecting ducts of kidney tubules and ADH acts to open water channels found here. Remember that the collecting ducts pass through the inner medulla of the kidney – a region of high osmolality. If water channels in the walls of the collecting ducts open, water will move out of the collecting duct into the medullary interstitium and finally into the circulation. The fall in plasma osmolality then leads to a slowing-down of ADH secretion by negative feedback (Figure 2.9).
Figure 2.9 Negative-feedback loop for antidiuretic hormone (ADH) release and control of plasma osmolality.
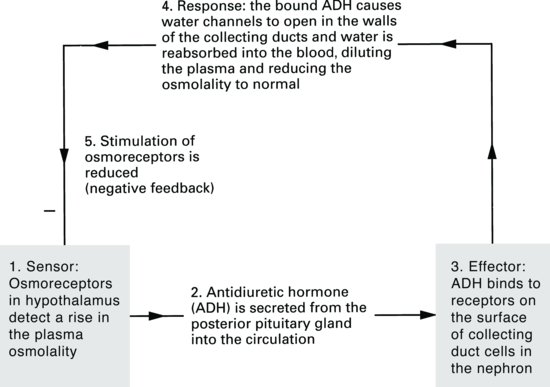
Aldosterone
Aldosterone is a steroid hormone secreted from the adrenal cortex. It has its effect on the distal tubule of the nephron: the more aldosterone is secreted, the more sodium is reabsorbed. Aldosterone, however, is not the sole determinant of body sodium balance, although in extreme cases of oversecretion of aldosterone, such as in Cushing’s disease, hypertension may result from over-retention of sodium and water.
The secretion of aldosterone, unlike ADH, is not directly triggered by extracellular osmolality, but is regulated by a peptide, angiotensin II. The function of this peptide is described in the section on the renin–angiotensin system, below.
Atrial natriuretic peptide
This peptide is released from cardiac atrial cells in response to an increased atrial stretch. It has five known major effects:
- inhibition of aldosterone secretion by the adrenal cortex;
- reduction of renin release by the kidney;
- reduction of ADH release from the posterior pituitary;
- vasodilation;
- natriuresis and diuresis.
All of these effects result in the excretion of sodium and water through the kidney, reducing the extracellular fluid volume back to normal. Other factors which may be important in sodium regulation are renal prostaglandins, kinins and the renal nerves. Failure to regulate the extracellular fluid volume occurs commonly in people with renal disease and leads to hypertension.
The renin–angiotensin-aldosterone pathway
The distal convoluted tubule forms a unique contact with the glomerulus, passing in the angle between the afferent and efferent arterioles. At this point, specialised cells called the macula densa in the wall of the distal tubule make contact with cells in the endothelium of the arterioles which release a hormone called renin. The macula densa and the renin-releasing cells are collectively called the juxtaglomerular apparatus or complex (see Figure 2.5).
The juxtaglomerular apparatus is responsible for maintaining a constant blood flow through the glomerulus and thus a constant GFR despite fluctuations in arterial pressure. This is achieved through a tubule feedback mechanism in which a fall in GFR results in a fall in the chloride ion concentration in the distal tubule. This stimulates the macula densa cells which send ‘signals’ to the renin-secreting cells to release renin. Renin acts via the renin–angiotensin system (Figure 2.10) to produce both local vasoconstriction of the efferent arteriole (increasing GFR) and peripheral vasoconstriction to increase arterial blood pressure. In addition, salt and water retention increase due to the effects of aldosterone.
Figure 2.10 The renin-angiotensin-aldosterone pathway.
Source: Tortora and Derrickson (2007), Introduction to the Human Body, The Essentials of Anatomy and Physiology, 7th edition. This material is reproduced with permission of John Wiley & Sons, Inc.
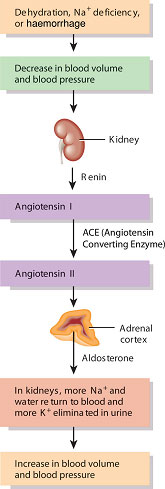
Aldosterone and the control of body potassium content
Potassium is the main intracellular cation. Only about 2% of the total body potassium content is extracellular. Potassium ions are freely filtered at the glomerulus: 65% of reabsorption takes place in the proximal tubule. However, the renal handling of potassium ions is not as efficient as that of sodium, nor is potassium conserved so well when there is a shortage. The secretion of potassium is also linked to that of sodium and hydrogen ions. Unlike sodium regulation, where aldosterone is just one factor in the regulation of sodium content, aldosterone is the only hormone involved in the control of potassium content and thus has a very important regulatory role. Small increases in extracellular potassium concentration directly stimulate aldosterone secretion from the adrenal cortex. The effect of aldosterone in the distal tubule of the nephron is to increase the secretion of potassium into the urine. When aldosterone levels fall, the reverse occurs and less potassium is secreted. The release of aldosterone stimulated by rises in extracellular potassium concentration is strongly controlled by a negative feedback system. Once the potassium concentration returns to normal the stimulus to secrete aldosterone is quickly switched off.
The hormone aldosterone increases sodium reabsorption in exchange for potassium or hydrogen ions. If excess potassium ions need to be secreted, then fewer hydrogen ions can be secreted and vice versa. Clinically, this phenomenon results in an association between metabolic acidosis and hyperkalaemia (or conversely, metabolic alkalosis and hypokalaemia), because in patients with acidosis the distal tubules will increase the rate of hydrogen ion secretion (to prevent a fall in plasma pH) by reducing the rate of potassium ion secretion, resulting in the retention of potassium ions in the blood, leading to hyperkalaemia.
Hypokalaemia and hyperkalaemia
Potassium is important in maintaining the membrane potential of nerve and muscle cells and hence affects their excitability. Disturbances in potassium balance show their effects in abnormal nerve and muscle function and may be life threatening.
Changes in muscle tone and in the electrocardiogram (ECG) may indicate an altered potassium balance. The diet almost invariably contains adequate potassium, so hypokalaemia generally occurs due to excessive losses. Persistent vomiting, diarrhoea, or the use of certain prescribed diuretics are the most likely causes of low blood potassium. Dialysis using an inappropriate concentration of potassium in the dialysate may also result in hypokalaemia. Treatment of hypokalaemia by the administration of a potassium salt must be undertaken with care since this may result in hyperkalaemia. The uptake of potassium into cells is also dependent on the acid–base balance in the patient. Acidosis (low blood pH) causes the release of potassium from cells so acid–base disturbances must first be corrected.
Hyperkalaemia can occur as a result of decreased potassium excretion in the renal patient. Insulin promotes uptake of potassium into cells, so insulin deficiency may lead to hyperkalaemia, as can β-blockade (Laing 2011) and excessive tissue breakdown following trauma.
Regulation of calcium, phosphate and magnesium
Calcium and phosphate are the main mineral constituents of bone and thus the majority of calcium and phosphate in the body is found in the skeleton. However, small amounts of both these ions are found in extracellular fluid. Calcium exists in the plasma in two forms. Approximately 50% exists in the free ionised form (1.25 mmol/l), and the other 50% in a bound form, mainly bound to protein, particularly albumin (1.25 mmol/l). Usually when serum calcium levels are measured, the total calcium concentration is measured (2.5 mmol/l). This total calcium is then ‘corrected’ depending on the blood albumin level, since it is the ionised form of the calcium that is important in the extracellular fluid in controlling nerve and muscle conduction. Any condition which leads to a fall in the ionised calcium concentration (even if total calcium remains normal) will lead to the classic symptoms of hypocalcaemia – tetany, muscle cramps and even convulsions. In situations of hypercalcaemia, the main effects seen are pruritus, extraskeletal calcification, renal calculi, peptic ulceration and changes in mental function such as memory loss and depression.
Inorganic phosphate (i.e. those ions carrying a charge) exists in several forms – ‘acid’ phosphate (H2PO4–) and ‘alkaline’ phosphate HPO4–. The normal plasma range of total inorganic phosphate is 0.87–1.45 mmol/l. Phosphate is important in buffer systems to maintain the plasma pH and exists in equilibrium with calcium.
Both calcium and phosphate are freely filtered at the glomerulus. When the plasma phosphate level is below 1 mmol/l, all the filtered phosphate is reabsorbed in the early proximal tubule. However, once the plasma phosphate level rises above 1 mmol/l, the amount of phosphate excreted in the urine rises in proportion to the plasma concentration. Further excretion of phosphate in the distal tubule (by secretion) can occur in response to the rise in the circulating level of PTH. Calcium reabsorption is very similar to that of sodium, in that approximately 65% occurs in the proximal tubule, and a further 20–25% in the ascending limb of the loop of Henle, leaving around 10–12% of filtered calcium being delivered to the distal tubule. How much more calcium is reabsorbed in the distal tubule depends on the levels of circulating PTH.
Magnesium is an important intracellular cation involved in energy storage and production. In all, 55% of total body magnesium is found within bones, so it is not surprising that magnesium balance is linked to that of calcium.
Parathyroid hormone increases tubular reabsorption of magnesium. Under normal circumstances, gastrointestinal absorption and urinary excretion of magnesium are equal. Gastrointestinal disorders may therefore decrease magnesium uptake but this is matched by a decrease in renal excretion. Most diuretics, and alcohol, increase renal magnesium excretion.
The renal handling of calcium and phosphate – the roles of PTH, vitamin D and calcitonin
The mechanisms by which plasma calcium and phosphate are regulated are closely interrelated. The two major regulators are PTH and vitamin D. Calcium and phosphate can enter the plasma from the gut and from the bone. Indeed almost all of the approximately 1 kg of calcium in a 70 kg person is found in the bone. Of this, a few grams exchange daily with the plasma calcium pool. Calcium and phosphate can leave the plasma by being redeposited in bone, or by renal excretion.
PTH is secreted by the parathyroid glands situated next to the thyroid. Secretion is stimulated by a decrease in plasma calcium concentration, and reduced when plasma calcium levels rise. Its effect is to raise plasma calcium concentration, mainly by increasing bone breakdown (resorption), releasing calcium ions. PTH also acts in the kidney, stimulating one step in the conversion of vitamin D to its active metabolite, calcitriol or 1,25-dihydroxycholecalciferol, also known as 1,25-hydroxyvitamin D.
Vitamin D is a steroid that enters the body either through dietary intake or by the effect of sunlight on the skin. However, this is an inactive form of the vitamin called cholecalciferol. To become activated it needs to undergo two metabolic conversions, one in the liver and one in the kidney. These conversions result in the formation of calcitriol. This active form has its effect in the gut, kidney and (in the presence of PTH) on bone, raising plasma calcium levels. The actions of vitamin D are summarised in Figure 2.11.
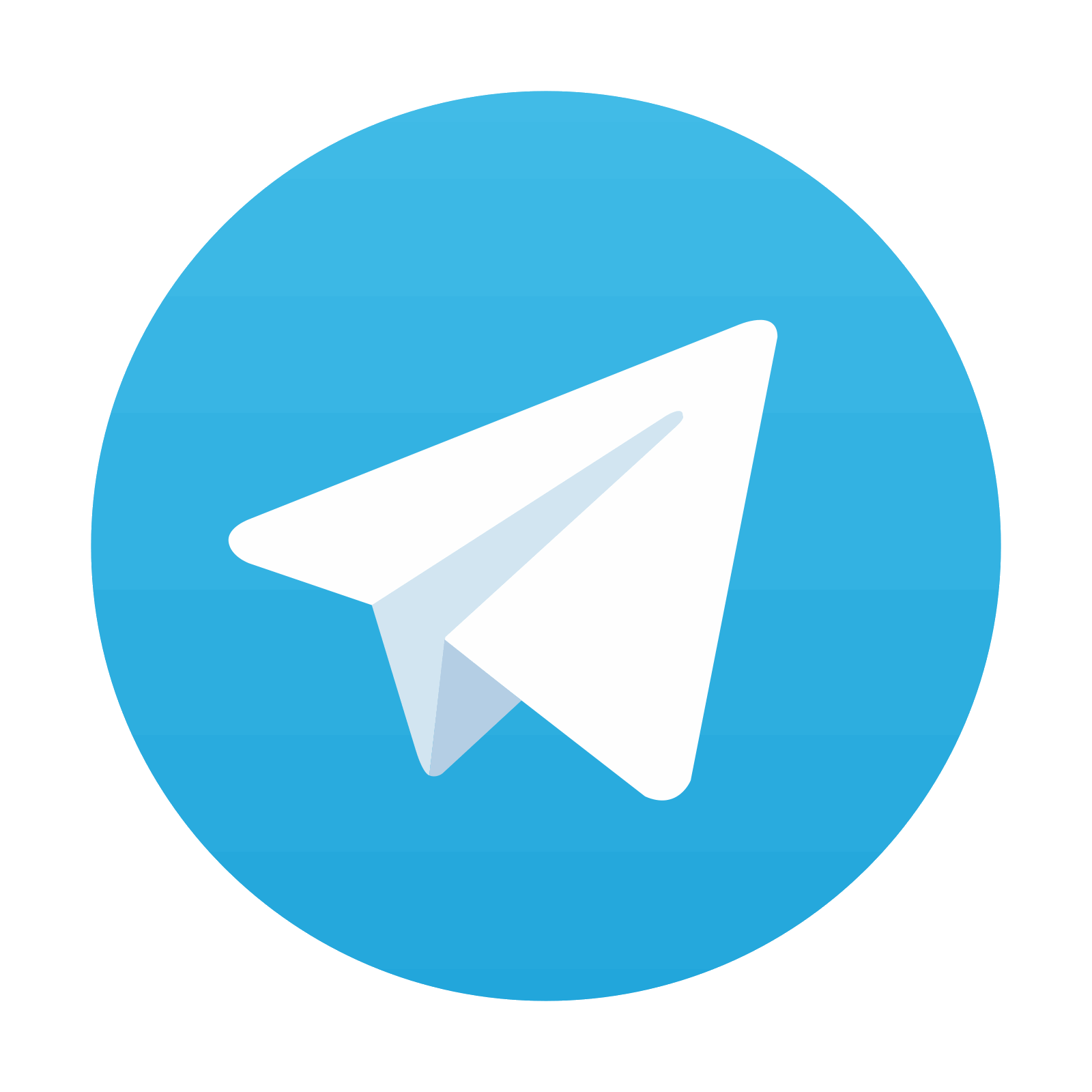
Stay updated, free articles. Join our Telegram channel
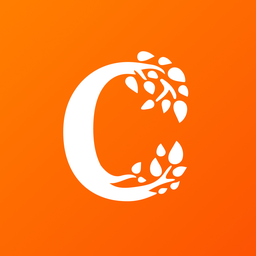
Full access? Get Clinical Tree
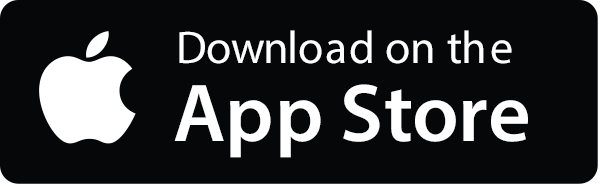
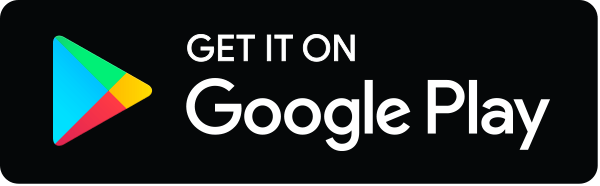