CHAPTER 2 a. The pulmonary system exists for the purpose of gas exchange. Oxygen (O2) and carbon dioxide (CO2) are exchanged between the atmosphere and the alveoli, between the alveoli and pulmonary capillary blood, and between the systemic capillary blood and all body cells. b. Atmospheric O2 is consumed by the body through cellular aerobic metabolism, which supplies the energy for life c. CO2, a by-product of aerobic metabolism, is eliminated primarily through lung ventilation d. The respiratory circuit includes all structures and processes involved in the transfer of O2 between room air and the individual cell, and the transfer of CO2 between the cell and room air e. Cellular respiration cannot be directly measured but is estimated by the amount of CO2 produced ( f. Exchange of O2 and CO2 at the alveolar-capillary level (external respiration) is called the respiratory exchange ratio (R). This is the ratio of the CO2 produced to the O2 taken up per minute. In homeostasis, the respiratory exchange ratio is the same as the respiratory quotient, 0.8. g. Proper functioning of the respiratory circuit requires efficient interaction of the respiratory, circulatory, and neuromuscular systems h. In addition to its primary function of O2 and CO2 exchange, the lung also carries out metabolic and endocrine functions as a source of hormones and a site of hormone metabolism. In addition, the lung is a target of hormonal actions by other endocrine organs. 2. Steps in the gas exchange process a. Step 1—Ventilation: Volume change, or the process of moving air between the atmosphere and the alveoli and distributing air within the lungs to maintain appropriate concentrations of O2 and CO2 in the alveoli i. Structural components involved in ventilation (1) Anatomic divisions: Right lung (three lobes—upper, middle, lower); left lung (two lobes—upper, lower). Lobes are divided into bronchopulmonary segments (ten right, nine left). Bronchopulmonary segments are subdivided into secondary lobules. (2) Lobule: Smallest gross anatomic units of lung tissue; contain the primary functional units of the lung (terminal bronchioles, alveolar ducts and sacs, pulmonary circulation). Lymphatics surround the lobule, keep the lung free of excess fluid, and remove inhaled particles from distal areas of the lung. (3) Bronchial artery circulation: Systemic source of circulation for the tracheobronchial tree and lung tissue down to the level of the terminal bronchiole. Alveoli receive their blood supply from the pulmonary circulation. (b) Conducting airways: Entire area from the nose to the terminal bronchioles where gas flows, but is not exchanged, is called anatomic dead space (Vdanat). Amount is approximately 150 ml but varies with patient size and position. Airways are a series of rapidly branching tubes of ever-diminishing diameter that eventually terminate in the alveoli. a) Serves as a passageway for air movement into the lungs b) Preconditions air by the action of the cilia, mucosal cells, and turbinate bones 1) Warms air to within 2° to 3° of body temperature; humidifies it to full saturation before it reaches the lower trachea 2) Filters by trapping particles larger than 6 μm in diameter (2) Pharynx: Posterior to nasal cavities and mouth a) Separation of food from air is controlled by local nerve reflexes b) Opening of eustachian tube regulates middle ear pressure (3) Larynx: Complex structure consisting of incomplete rings of cartilage and numerous muscles and ligaments a) Vocal cords: Speech function 1) Narrowest part of the conducting airways in adults 2) Contraction of muscles of the larynx causes the vocal cords to change shape 3) Vibration of the vocal cords produces sound. Speech is a joint function of the vocal cords, lips, tongue, soft palate, and respiration, with control by temporal and parietal lobes of the cerebral cortex. b) Valve action by the epiglottis helps to prevent aspiration c) Cough reflex: Cords close and intrathoracic pressure increases to permit coughing or Valsalva maneuver (4) Trachea: Tubular structure consisting of 16 to 20 incomplete, or C-shaped, cartilaginous rings that stabilize the airway and prevent complete collapse with coughing a) Begins the conducting system, or tracheobronchial tree c) Mucosal cells trap foreign material d) Cilia propel mucus upward through the airway e) Cough reflex present, especially at the point of tracheal bifurcation (carina) f) Smooth muscle innervated by the parasympathetic branch of the autonomic nervous system (5) Major bronchi and bronchioles a) Smooth muscle walls (no cartilage); bronchospasm may narrow the lumen and increase airway resistance b) Ciliated mucosal cells become flattened, with progressive loss of cilia toward the alveoli c) Sensitive to CO2 levels: Increased levels induce bronchiolar dilation, decreased levels induce constriction (c) Gas exchange airways: Semipermeable membrane permits the movement of gases according to pressure gradients. These airways are not major contributors to airflow resistance but do contribute to the distensibility of the lung. The acinus (terminal respiratory unit) is composed of the respiratory bronchiole and its subdivisions (Figure 2-1). (1) Respiratory bronchioles and alveolar ducts a) Most important structures in gas exchange b) Alveolar surface area is large and depends on body size. Total surface area is about 70 m2 in a normal adult. Thickness of the respiratory membrane is about 0.6 μm. This fulfills the need to distribute a large quantity of perfused blood into a very thin film to ensure near equalization of O2 and CO2. 1) Type I: Squamous epithelium, adapted for gas exchange, sensitive to injury by inhaled agents, structured to prevent fluid transudation into the alveoli 2) Type II: Large secretory, highly active metabolically; origin of surfactant synthesis and type I cell genesis 1) Phospholipid monolayer at the alveolar air-liquid interface; able to vary surface tension with alveolar volume 2) Enables surface tension to decrease as alveolar volume decreases during expiration, which prevents alveolar collapse 3) Decreases the work of breathing, permits the alveoli to remain inflated at low distending pressures, reduces net forces causing tissue fluid accumulation 4) Reduction of surfactant makes lung expansion more difficult; the greater the surface tension, the greater the pressure needed to overcome it 5) Surfactant also detoxifies inhaled gases and traps inhaled and deposited particles e) Alveolar-capillary membrane (alveolar epithelium, interstitial space, capillary endothelium) 1) Bathed by interstitial fluid; lines the respiratory bronchioles, alveolar ducts, and alveolar sacs; forms the walls of the alveoli 2) About 1 μm or less thick (less than one red blood cell); permits rapid diffusion of gases; any increase in thickness diminishes gas diffusion 3) Total surface area of about 70 m2 in an adult is in contact with about 60 to 140 ml of pulmonary capillary blood at any one time f) Gas exchange pathway (Figure 2-2): Alveolar epithelium → alveolar basement membrane → interstitial space → capillary basement membrane → capillary endothelium → plasma → erythrocyte membrane → erythrocyte cytoplasm ii. Alveolar ventilation ( (a) Alveolar ventilation is one component of minute ventilation (1) Minute ventilation ( VT × RR = Tidal volume is easily measured at the bedside by hand-held devices or a mechanical ventilator. Exhaled minute ventilation is a routinely measured parameter for patients on ventilators. (2) Minute ventilation is composed of both alveolar ventilation ( where (3) Ratio of dead space to tidal volume (VD/VT) is measured to determine how much of each breath is wasted (i.e., does not contribute to gas exchange). Normal values for spontaneously breathing patients range from 0.2 to 0.4 (20% to 40%). (b) Alveolar ventilation cannot be measured directly; it is inversely related to arterial CO2 pressure (Paco2) in a steady state by the following formula: (c) Since (d) Paco2 is the only adequate indicator of effective matching of alveolar ventilation to metabolic demand. To assess ventilation, Paco2 must be measured. (e) If Paco2 is low, alveolar ventilation is high; hyperventilation is present (f) If Paco2 is within normal limits, alveolar ventilation is adequate (g) If Paco2 is high, alveolar ventilation is low and hypoventilation is present iii. Defense mechanisms of the lung (a) Although an internal organ, the lung is unique in that it has continuous contact with particulate and gaseous materials inhaled from the external environment. In the healthy lung, defense mechanisms successfully defend against these natural materials by the following means: (1) Structural architecture of the upper respiratory tract, which reduces deposited and inhaled materials (2) Processing system, including respiratory tract fluid alteration and phagocytic activity (3) Transport system, which removes material from the lung (4) Humoral and cell-mediated immune responses, which may be the most important bronchopulmonary defense mechanisms (b) Loss of normal defense mechanisms may be precipitated by disease, injury, surgery, insertion of an endotracheal tube, or smoking (c) Upper respiratory tract warms and humidifies inspired air, absorbs selected inhaled gases, and filters out particulate matter. Soluble gases and particles larger than 10 μm are aerodynamically filtered out. Normally, no bacteria are present below the larynx. (d) Inhaled and deposited particles reaching the alveoli are coated by surface fluids (surfactant and other lipoproteins) and are rapidly phagocytized by pulmonary alveolar macrophages (e) Macrophages and particles are transported in mucus by bronchial cilia, which beat toward the glottis and move materials in a mucus-fluid layer, eventually to be expectorated or swallowed. This process is referred to as the mucociliary escalator. Pulmonary lymphatics also drain and transport some cells and particles from the lung. (f) Antigens activate the humoral and cell-mediated immune systems, which add immunoglobulins to the surface fluid of the alveoli and activate alveolar macrophages (g) Disruption of or injury to these defense mechanisms predisposes to acute or chronic pulmonary disease (a) Muscles of respiration: Act of breathing is accomplished through muscular actions that alter intrapleural and pulmonary pressures and thus change intrapulmonary volumes (1) Muscles of inspiration: During inspiration, the chest cavity enlarges. This enlargement is an active process brought about by the contraction of the following: a) Diaphragm: Major inspiratory muscle 1) Normal quiet breathing is accomplished almost entirely by this dome-shaped muscle, which divides the chest from the abdomen 2) Divided into two “leaves”—the right and left hemidiaphragms 3) Downward contraction increases the superior-inferior diameter of the chest and elevates the lower ribs 4) Innervation is from the C3 to C5 level through the phrenic nerve 5) Normally, accounts for 75% of tidal volume during quiet inspiration 6) Facilitates vomiting, coughing and sneezing, defecation, and parturition b) External intercostal muscles 1) Increase the anterior-posterior (A-P) diameter of the thorax by elevating the ribs 2) A-P diameter is about 20% greater during inspiration than during expiration c) Accessory muscles in the neck: Scalene and sternocleidomastoid (2) Muscles of expiration: During expiration, the chest cavity decreases in size. This is a passive act unless forced, and the driving force is derived from lung recoil. Muscles used when increased levels of ventilation are needed are the following: (b) Pressures within the chest: Movement of air into the lungs requires a pressure difference between the airway opening and alveoli sufficient to overcome the resistance to airflow of the tracheobronchial tree (Table 2-1) (1) Air flows into the lungs when intrapulmonary air pressure falls below atmospheric pressure (2) Air flows out of the lungs when intrapulmonary air pressure exceeds atmospheric pressure (3) Intrapleural pressure is normally negative with respect to atmospheric pressure as a result of the elastic recoil of the lungs, which tend to pull away from the chest wall. This “negative” pressure prevents the collapse of the lungs. (4) Increased effort (forced inspiration or expiration) may produce much greater changes in intrapulmonary and intrapleural pressures during inspiration and expiration (c) Structural components of the thorax (1) For protection: Sternum, spine, ribs a) Visceral layer next to the lungs; parietal layer next to the chest wall b) Pleural fluid between layers: Allows smooth movement of the visceral layer over the parietal layer c) Adherence: Pleural space is normally a potential space (vacuum); because of a constant negative pressure (less than atmospheric pressure by 4 to 8 mm Hg), any change in the volume of the thoracic cage is reflected by a similar change in the volume of the lungs d) Nerve supply: Parietal pleura has fibers for pain transmission, but visceral pleura does not (1) Elastic resistance (static properties) a) Lung, if removed from the chest, collapses to a smaller volume because of lung elastic recoil. This tendency of the lungs to collapse is normally counteracted by the chest wall tendency to expand. Volume of air in the lungs depends on the equal and opposite balance of these forces. b) Compliance (CL) is an expression of the elastic properties of the lung and is the change in volume (ΔV) accomplished by a change in pressure (ΔP): If compliance is high, the lung is more easily distended; if compliance is low, the lung is stiff and more difficult to distend (2) Flow resistance (dynamic properties) a) Airway resistance must be overcome to generate flow through the airways b) Changes in airway caliber affect airway resistance. Examples are changes caused by bronchospasm or secretions. c) Flow through the airway depends on pressure differences between the two ends of the tube as well as resistance. Driving pressure for flow in airways is the difference between atmospheric and alveolar pressures. v. Control of ventilation: Although the process of breathing is a normal rhythmic activity that occurs without conscious effort, it involves an intricate controlling mechanism within the central nervous system (CNS). Basic organization of the respiratory control system is outlined in Figure 2-3. (a) Respiratory generator: Located in the medulla and composed of two groups of neurons (1) One group initiates respiration and regulates its rate (2) One group controls the “switching off” of inspiration and thus the onset of expiration (b) Input from other regions of the CNS (1) Pons: Input is necessary for normal, coordinated breathing (2) Cerebral cortex: Exerts a conscious or voluntary control over ventilation (c) Chemoreceptors: Contribute to a feedback loop that adjusts respiratory center output if blood gas levels are not maintained within the normal range (1) Central chemoreceptors: Located near the ventrolateral surface of the medulla (but are separate from the medullary respiratory center) a) Respond not directly to blood partial pressure of carbon dioxide (Pco2) but, rather, to the pH of the extracellular fluid (ECF) surrounding the chemoreceptor b) Feedback loop for CO2 can be summarized as follows: Increased arterial Pco2 → increased brain ECF Pco2 → decreased brain ECF pH → decreased pH at chemoreceptor → stimulation of central chemoreceptor → stimulation of medullary respiratory center → increased ventilation → decreased arterial Pco2 (2) Peripheral chemoreceptors: Located in the carotid body and aortic body (1) Stretch receptors in the bronchial wall respond to changes in lung inflation (Hering-Breuer reflex) (2) Irritant receptors in the lining of the airways respond to noxious stimuli, such as irritating dust and chemicals (3) “J” (juxtacapillary) receptors in the alveolar interstitial space a) Cause rapid shallow breathing in response to deformation from increased interstitial volume due to high pulmonary capillary pressures (such as in heart failure or inflammation) b) Stimulation can also cause bradycardia, hypotension, and expiratory constriction of the glottis (4) Receptors in the chest wall (in the intercostal muscles) b. Step 2—Diffusion: Process by which alveolar air gases are moved across the alveolar-capillary membrane to the pulmonary capillary bed and vice versa. Diffusion occurs down a concentration gradient from a higher to a lower concentration. No active metabolic work is required for the diffusion of gases to occur. Work of breathing is accomplished by the respiratory muscles and the heart, which produce a gradient across the alveolar-capillary membrane. i. Ability of the lung to transfer gases is called the diffusing capacity of the lung (DL). Diffusing capacity measures the amount of gas (O2, CO2, carbon monoxide) diffusing between the alveoli and pulmonary capillary blood per minute per millimeter Hg mean gas pressure difference. ii. CO2 is 20 times more diffusible across the alveolar-capillary membrane than O2. If the membrane is damaged, its decreased capacity for transporting O2 into the blood is usually more of a problem than its decreased capacity for transporting CO2 out of the body. Thus, the diffusing capacity of the lungs for O2 is of primary importance. iii. Diffusion is determined by several variables: (a) Surface area available for gas exchange (b) Integrity of the alveolar-capillary membrane (c) Amount of hemoglobin (Hb) in the blood (d) Diffusion coefficient of gas as well as contact time (e) Driving pressure: Difference between alveolar gas tensions and pulmonary capillary gas tensions (Table 2-2). This is the force that causes gases to diffuse across membranes. TABLE 2-2 (1) During the breathing of 100% O2, the alveolar O2 tension (PAo2) becomes so large that the difference between PAo2 and P (2) Therefore, hypoxemia due solely to diffusion defects is usually improved by breathing 100% O2 iv. A–a gradient (PAo2 − Pao2) is the alveolar to arterial O2 pressure difference (i.e., the difference in the partial pressure of O2 in the alveolar gas spaces [PAo2] and the pressure in the systemic arterial blood [Pao2]). This gradient is always a positive number. (a) Normal gradient in young adults is less than 10 mm Hg (on room air) but increases with age and may be as high as 20 mm Hg in people over age 60 years (b) A–a gradient provides an index of how efficient the lung is in equilibrating pulmonary capillary O2 with alveolar O2. It indicates whether gas transfer is normal. (c) Large A–a gradient generally indicates that the lung is the site of dysfunction (except with cardiac right-to-left shunting) (d) Formula for calculation (on room air) where 47 = vapor pressure of water at 37° C (in mm Hg) PIo2 = pressure of inspired O2 0.8 = assumed respiratory quotient (ratio of CO2 produced to O2 consumed per unit time) Pb = barometric pressure (sea level normal Pb is 760 mm Hg) Therefore, FIo2 (Pb − 47) − (Paco2 ÷ 0.8) − Pao2 = A–a gradient Example of calculation: 0.21 (760 − 47) − (40 ÷ 0.8) − 90 = 10 (e) Normally, A–a gradient increases with age and increased FIo2 (f) Pathologic conditions that increase the A–a gradient (difference) include the following: c. Step 3—Transport of gases in the circulation i. Approximately 97% of O2 is transported in chemical combination with Hb in the erythrocyte and 3% is carried dissolved in the plasma. Pao2 is a measurement of the O2 tension in the plasma and is a reflection of the driving pressure that causes O2 to dissolve in the plasma and combine with Hb. Thus, O2 content is related to Pao2. ii. Oxyhemoglobin dissociation curve (Figure 2-4) (a) Relationship between O2 saturation (and content) and Pao2 is expressed in an S-shaped curve that has great physiologic significance. It describes the ability of Hb to bind O2 at normal Pao2 levels and release it at lower Po2 levels. (b) Relationship between the content and pressure of O2 in the blood is not linear (1) Upper flat portion of the curve is the arterial association portion. Dissociation relationship in this range protects the body by enabling Hb to retain high saturation with O2 despite large decreases (down to 60 mm Hg) in Pao2. (2) Lower steep portion of the curve is the venous dissociation portion. Dissociation relationship in this range protects the body by enabling the tissues to withdraw large amounts of O2 with small decreases in Pao2. (c) Hb O2 binding is sensitive to O2 tension. The binding is reversible; the affinity of Hb for O2 changes as Po2 changes. (1) When Po2 is increased (as in pulmonary capillaries), O2 binds readily with Hb (2) When Po2 is decreased (as in tissues), O2 unloads from Hb (d) Increase in the rate of O2 utilization by tissues causes an automatic increase in the rate of O2 release from Hb (e) Shifts of the oxyhemoglobin curve (1) Shifts to the right: More O2 is unloaded for a given Po2, which thus increases O2 delivery to the tissues. These shifts are caused by the following: (2) Shifts to the left: O2 is not dissociated from Hb until tissue and capillary O2 are very low, which thus decreases O2 delivery to the tissues. These shifts are caused by the following: (3) 2,3-DPG is an intermediate metabolite of glucose that facilitates the dissociation of O2 from Hb at the tissues. Decreased levels of 2,3-DPG impair O2 release to the tissues. This may occur with massive transfusions of 2,3-DPG–depleted blood and anything that decreases phosphate levels. iii. Ability of Hb to release O2 to the tissues is commonly assessed by evaluating the P50 (a) P50 = the partial pressure of O2 at which the Hb is 50% saturated, standardized to a pH of 7.40 (b) Normal P50 is about 26.6 mm Hg; varies with the disease process iv. Each gram of normal Hb can maximally combine with 1.34 ml of O2 when fully saturated (values of 1.36 or 1.39 are sometimes used) v. Amount of O2 transported per minute in the circulation is a factor of both the arterial O2 concentration (Cao2) and cardiac output. This amount reflects how much O2 is delivered to tissues per minute and is dependent on the interaction of the circulatory system (delivery of arterial blood), erythropoietic system (Hb in red blood cells), and respiratory system (gas exchange) according to the following equations: (a) O2 content (Cao2) is calculated from O2 saturation, O2 capacity, and dissolved O2 (1) O2 capacity is the maximal amount of O2 the blood can carry. It is expressed in milliliters of O2 per deciliter (100 ml) of blood (ml/dl) and is calculated by multiplying Hb in grams by 1.34. (2) O2 saturation is the percentage of Hb actually saturated with O2 (Sao2 or S (3) O2 content is the actual amount of O2 the blood is carrying (oxyhemoglobin plus dissolved O2) O2 content = (O2 capacity × O2 saturation) + (0.0031 × Pao2) ml/min = arterial O2 content (ml/dl) × cardiac output (L/min) × 10 (conversion factor) vi. Focusing only on the O2 tension of the blood is unwise because an underestimation of the severity of hypoxemia may result. O2 content and transport are more reliable parameters because they take into account the Hb concentration and cardiac output. vii. Arterial–mixed venous differences in O2 content (Cao2 − C (a) Of the 1000 to 1200 ml of O2 delivered per minute to tissues, cells typically use only about 250 to 300 ml ( (b) Normal Cao2 − C (Hb × 1.34) (Sao2 − S (c) Fall in C (d) These are average values; actual O2 utilization is different for different tissues. The heart uses almost all the O2 it receives. viii. CO2 transport: CO2 is carried in the blood in three forms, as follows: (a) Physically dissolved (Paco2), which accounts for 7% to 10% of CO2 transported in the blood (b) Chemically combined with Hb as carbaminohemoglobin. This reaction occurs rapidly, and reduced Hb can bind more CO2 than oxyhemoglobin. Thus, unloading of O2 facilitates loading of CO2 (Haldane effect) and accounts for about 30% of CO2 transport. (c) As bicarbonate (HCO3−) through a conversion reaction: where CA = carbonic anhydrase (1) Reaction accounts for 60% to 70% of CO2 in the body (2) Reaction is slow in plasma and fast in red blood cell owing to the CA enzyme (3) When the concentration of these ions increases in red blood cells, HCO3− diffuses but H+ remains (4) To maintain electrical neutrality, chloride diffuses from the plasma (the “chloride shift”) ix. Pulmonary circulation (pulmonary artery, arterioles, capillary network, venules, and veins) (a) Pulmonary vessels are peculiarly suited to maintaining a delicate balance of flow and pressure distribution that optimizes gas exchange. They are richly innervated by the sympathetic branch of the autonomic nervous system. (b) In contrast to the systemic circulation, the pulmonary circulation is a low-resistance system. Pulmonary arteries have far thinner walls than systemic arteries do, and vessels distend to allow for increases in volume from systemic circulation. Intrapulmonary blood volume increases or decreases of approximately 50% occur with changes in the relationship between intrathoracic and extrathoracic pressure. (c) Pulmonary arteries accompany the bronchi within the lung and give rise to a rich capillary network within the alveolar walls. Pulmonary veins are not contiguous with the bronchial tree. (d) Primary function of the pulmonary circulation is to act as a transport system (1) Transport of blood through the lung a) Flow resistance through vessels (R) is defined by Ohm’s law: where ΔP = the pressure difference between the two ends of the vessel (upstream and downstream pressures) Driving pressure for flow in the pulmonary circulation is the difference between the inflow pressure in the pulmonary artery and the outflow pressure in the left atrium b) In the lung, measurement of flow resistance is pulmonary vascular resistance (PVR) c) About 12% of the total blood volume of the body is in the pulmonary circulation at any given time d) Normal pressures in the pulmonary vasculature 1) Mean pulmonary artery pressure: 10 to 15 mm Hg 2) Mean pulmonary venous pressure: 4 to 12 mm Hg 3) Mean pressure gradient: Approximately 10 mm Hg (considerably less than the systemic gradient) 4) Pressures are higher at the base of the lung than at the apex e) Unique characteristic of the pulmonary arterial bed is that it constricts in response to hypoxia. Diffuse alveolar hypoxia causes generalized vasoconstriction, which results in pulmonary hypertension. Localized hypoxia causes localized vasoconstriction that does not increase pulmonary hypertension. This localized vasoconstriction directs blood away from poorly ventilated alveoli and thus improves overall gas exchange. f) Chronic pulmonary hypertension (increased PVR) can result in right ventricular hypertrophy (cor pulmonale) 1) Transvascular transport of fluids and solutes a) Transvascular fluid filtration in the lung (and all other organs) is described by the Starling equation. This means that fluid and solutes move due to increases or decreases in hydrostatic or osmotic filtration pressures or due to changes in the permeability of vessel walls to fluids or proteins. b) Thus, excess fluid in the lung (pulmonary edema) can result from either a net increase in hydrostatic pressure forces (favoring filtration) or a decreased resistance to filtration a) All cardiac output passes through the lung before reaching systemic circulation. Therefore, pulmonary circulation can influence the composition of the blood supplying all organs. b) Several humoral substances are added, extracted, or metabolized in the lung. Examples are inactivation of vasoactive prostaglandins, conversion of angiotensin I to angiotensin II, and inactivation of bradykinin. d. Step 4—Diffusion between the systemic capillary bed and body tissue cells i. Pressure gradients allow for the diffusion of O2 and CO2 among systemic capillaries, interstitial fluid, and cells (Figures 2-5 and 2-6) ii. Within the mitochondria of each individual cell, O2 is consumed through aerobic metabolism. This process produces the energy bonds of adenosine triphosphate and the waste products of CO2 and water. 3. Hypoxemia: Hypoxemia is a state in which the O2 pressure or saturation of O2 in arterial blood, or both, is lower than normal. Hypoxemia is generally defined as Pao2 less than 55 mm Hg or Sao2 below 88% at sea level in an adult breathing room air. Disorders that lead to hypoxemia do so through one or more of the following processes. i. Reduced ambient pressure (Pb) or reduced O2 concentration of inspired air (FIo2) ii. If the lungs are normal, the A–a gradient will be normal iii. Rarely a clinically important cause of arterial hypoxemia. Reduced PB occurs at high altitudes in healthy humans and in enclosed spaces such as mine cave-ins where fresh air is not replenished; FIo2 remains normal, however. b. Alveolar hypoventilation (increased Paco2) i. Decrease in alveolar ventilation from disorders of the respiratory center, peripheral nerves that supply the muscles of respiration, the respiratory muscles of the chest wall, or the lungs; medications that diminish ventilation ii. This causes an increase in Paco2, which results in a fall in PAo2 according to the alveolar air equation iii. If the lungs are normal, the A–a gradient will be normal. Hypoxemia will improve with ventilation.
The Pulmonary System
SYSTEMWIDE ELEMENTS
co2) and the amount of O2 consumed (
o2). Ratio of these two values is called the respiratory quotient. Respiratory quotient is normally about 0.8 but changes according to the nutritional substrate being burned (i.e., protein, fats, or carbohydrates). Patients fully maintained on intravenous (IV) glucose alone will have a respiratory quotient approaching 1.0 as a result of the metabolic end product, CO2.
A): That part of total ventilation taking part in gas exchange and, therefore, the only part useful to the body
E): Amount of air exchanged in 1 minute. Equal to exhaled tidal volume (VT) multiplied by respiratory rate (RR or f). Normal resting minute ventilation in an adult is about 6 L/min:
E (500 ml × 12 = 6000 ml)
A) and physiologic dead space ventilation (
D):
= volume of gas per unit of time
co2 remains the same in a steady state, measurement of the patient’s Paco2 reveals the status of the alveolar ventilation
o2 (mixed venous O2 tension) significantly increases, proportionately increasing the driving pressure
o2) and is usually measured directly. It is equal to the O2 content divided by the O2 capacity multiplied by 100.
o2) is the difference between arterial O2 content (Cao2) and mixed venous O2 content (C
o2) and reflects the actual amount of O2 extracted from the blood during its passage through the tissues
o2 or O2 consumption). If
o2 remains constant, changes in cardiac output can be related to changes in the Cao2 − C
o2 gradient or difference. Mixed venous O2 values are measured from the distal tip of pulmonary artery catheters.
o2 is 4.5 to 6 ml/dl
o2) + (Pao2 − P
o2) (0.0031)
o2 resulting in a rise in the Cao2 − C
o2 gradient signifies decreased cardiac output and inadequate tissue perfusion if
o2 is constant
Stay updated, free articles. Join our Telegram channel
Full access? Get Clinical Tree
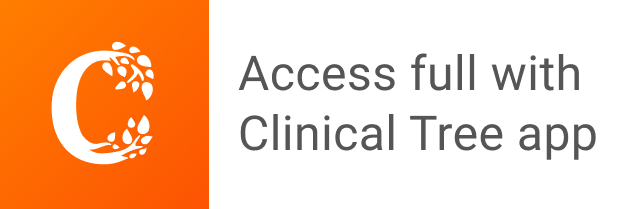