389CHAPTER 11
The Genetic Basis of Cancer
Gwen Anderson, Jennifer Francis, and Caitlyn Cornell
Cancers arise from a wide variety of etiologies. The incidence of most cancers increases significantly with age, which can be attributed, in part, to the accumulation of genetic alterations triggered by environmental exposures that collectively predispose a cell to inappropriate division, regulation, and function. It is important to understand that most cancers are multifactorial, and genetic insults contribute to a progressive compilation of events whereby somatic cell changes develop into an uncontrolled cancer growth. Amid these changes, the cells that develop into cancer have evolved over many divisions that can take years to progress and manifest into an unregulated, undifferentiated mass of tumor cells.
Cancers all share a common link through the entwinement of environmental, genetic, genomic, and epigenetic alterations that characterize unregulated cell growth and neoplastic transformation. With the mapping of the human genome in 2003 and the advent of next-generation sequencing technology, researchers and clinicians have a new understanding of cancer genetic expression profiles, molecular pathways that are potential targets for selective biotherapies, and cancer-specific tissue biomarkers that can be used for early diagnosis and surveillance of treatment effectiveness. In this chapter, genetics refers to the inherited germline gene mutations and chromosomal rearrangements that lead to cancer. Epigenetics refers to alterations in genes and chromosomes that can be inherited or acquired from the environment.
For the purpose of understanding the genomic etiology of cancer, an overview of four types of cancer genomic mechanisms is provided: hereditary, chromosomal, epigenetic biochemical modifications, and sporadic mutations. First, each type of mechanism is described followed by the genetic etiologies of specific pediatric and adult cancers. The genomic mechanisms for cancers are identified in a pediatric table and an adult table. The commonly occurring cancers in the United States have been selected to illustrate each of the genomic mechanisms in cancer.
GENOMIC MECHANISMS IN CANCER: AN OVERVIEW
Hereditary cancers involve specific genetic changes, passed from parent to child, which predispose an individual to cancer. Genetic testing can show how inherited single gene mutations and chromosomal rearrangements contribute to carcinogenesis. However, 390only 5% to 10% of all cancers can be attributed to a hereditary gene mutation. The fact that cancers recur in families is well documented in large population-based studies. By examining the family medical histories and degree of shared DNA among biological relatives, factors that predict risk and calculate statistical population risk of cancer occurrence and recurrence in families are identified. The vast majority of genetic alterations are spontaneously acquired during an individual’s lifetime. These initiate oncogenesis through the disruption of DNA repair mechanisms, silencing tumor suppressor genes and promoting oncogenes and oncoviruses that lead down a multistep pathway to cancer mutagenesis and tumor growth. Epigenetic mechanisms involve a chemical structure, the epigenome, which surrounds the DNA and further influences gene translation and expression in somatic cells.
Inherited Mutations
Some cancers arise due to an inherited predisposition, such as occurs in single gene mutations characteristic of inherited cancer syndromes. This type of cancer susceptibility begins as a genetic alteration in parental gametes (sperm and ova) and is then incorporated into the zygote during fertilization. Since an individual develops from a single-celled zygote, every somatic cell in the body, and the germ cells, will carry this germline mutation. Transmission of the mutation in the offspring’s germ cells is how a heritable predisposition to cancer is passed from one generation to the next; mutations arising in somatic cells are not involved in fertilization and, thus, are not passed on to the next generation. Note that it is the genetic alteration that increases risk for cancer that is inherited and not the cancer itself. In addition, the gene mutation is located in every cell of the body, not just in the cancer tissue or organ of interest.
Due to the number of roles that different gene products play within the body’s complex pathways, genes that have been identified in heritable cancers are frequently implicated in more than one cancer or condition. It is also important to understand that a single cancer can emerge from very distinct, separate pathways, and thus, different genes can contribute to the development of the same cancer through different mechanisms.
Hallmarks of inherited cancer syndromes include early age of onset, development of multiple cancers within a single individual, bilateral tumors in paired organs, cancer in the gender less frequently affected (such as breast cancer in males), and presentation of the same cancer or clusters of different types of cancers among biological relatives. Examples include hereditary breast and ovarian cancer syndrome (HBOC), Lynch syndromes I (HNPCC) and II, and familial adenomatous polyposis (FAP), as will be discussed in this chapter.
Inheritance of cancer-implicated genes does not imply obligate carcinogenesis, but it does increase one’s risk above that of the general population. People who have a gene mutation may never develop cancer, while other gene mutation carriers in the same family do develop the disease at an early age. The specific gene mutation variant and the pattern of inheritance are highly influential; the presence of one damaged allele may be sufficient (dominant) to exert an effect in one case, whereas both alleles (recessive) may be needed in another. Cancer predispositions associated with germline mutations can be transmitted as autosomal dominant, recessive, X-linked, 391or through maternal mitochondrial DNA, with the majority being autosomal dominant. Autosomal dominant transmissions pose a 50% risk of passing the mutation to each offspring. Lifestyle and environmental factors also play a contributing role; the vast majority of these syndromes, accounting for 5% to 10% of all cancers, require additional, acquired genetic insults in order for a predisposition to turn into active cancer. However, inherited predispositions still entail relatively high rates of penetrance. Early age of disease onset is a hallmark of inherited cancer gene mutations because people with heritable predispositions are already one step (or more) closer to acquiring the number of genetic insults needed to initiate carcinogenesis.
Chromosomal Rearrangements
With cancer development and progression in somatic cells and tissues, aberrations in chromosome structure and number are prevalent in many cancers and can contribute to increased susceptibility to cancer initiation at an early age as well as later in life. There are three basic types of chromosomal alterations that effect cancer etiology. One is an alteration in the copy number of chromosomes inside the nucleus of the cell. Abnormal numbers of chromosomes is termed aneuploid or amplification of chromosomal material. Copy number variation can lead to multiple oncogene copies or even the loss of important tumor suppressor genes. For example, inheritance of an extra chromosome increases the risk of cancer in people with Down syndrome.
Another mechanism is a translocation of one or more segments on one or more chromosomes to a new position on the same or a different chromosome. This type of chromosomal instability often results from errors during cell division in germline and somatic cells. Translocations can be balanced, where no additions or deletions take place and the same genomic material is retained inside the cell. Repositioning DNA within the genome may occur by the breakage of one or more chromosome segments and the subsequent incorrect reattachment to the same or a different chromosome. In one type of translocation, a coding region from one gene may be placed next to the promoter region of another gene. For example, translocation of a proto-oncogene to a particularly active promoter may incite hyperactivity and result in uncontrolled growth. This is the case in Burkitt lymphoma involving the movement of the MYC proto-oncogene on chromosomes 8 to the immunoglobulin heavy chain (IG) promoter on chromosome 14. Similarly, a translocation can be unbalanced, resulting in a loss or gain of genome material. In this instance, a translocation may cause the loss of an important tumor suppressor gene.
In another example, the translocation of two genes can produce a novel gene product through gene fusion, where two genes become one novel gene with a different structure and function. One example of this is with chronic myelogenous leukemia (CML) where two genes, BCR and ABL, fuse to become one new gene with aberrant properties. CML involves the side-by-side placement of two different gene coding regions, resulting in the production of a fusion gene with a novel BCR–ABL protein that activates growth pathways within the cell. Chromosomal translocations are one of many contributors to cancer through instigating oncogene activation or suppressing important protective mechanisms such as tumor suppression or DNA repair.
392It is important to remember that these same chromosomal aberrations take place inside somatic cells as neoplastic transformations take hold and progress. As cancer progresses, cells exhibit a higher frequency of both types of chromosomal aberrations (copy number variation and translocations) that further degrade the normal genomic structure and function of molecular pathways inside the cell. Structural instability of chromosomes leads to further impairments in the cell’s ability to repair DNA or recover from the slippery slope of tumorigenesis. Four types of genetic alterations disrupt cell differentiation and function: DNA repair, oncogenes, chromosomal translocations, and tumor suppressor genes (Figure 11.1)
Epigenetic Biochemical Modifications
In 1942, biologist Conrad Waddington defined epigenetics as “the branch of biology which studies the causal interactions between genes and their products, which bring the phenotype into being”. In modern times, the epigenome is known as a second inherited biological code that sits on the DNA genome and serves as a chemical modifier inside the chromatin structure of chromosomes. Epigenetic inheritance refers to cellular information that is inherited during cell division but is not encoded in the DNA sequence of the genome. The epigenome is a complex assortment of proteins and chemical modifications that are associated with DNA, control its transcription, and influence which genes are expressed and in which cells.
There is an abundance of research evidence, which indicates that epigenome chemical modifications are both inherited and acquired in response to environmental exposures, especially early in life. Epigenetic alterations affect the process of gene to protein expression, thus metabolic functioning within the cytoplasm of the cell. Alterations in gene regulation can disrupt cellular homeostasis during transcription, translation, or post-translation processes to effect gene signaling pathways, gene expression, and protein expression, thus affecting cellular functioning.
Acquired somatic cell epigenomic changes describe another genetic mechanism that leads to cancer development. There are numerous types of interrelated epigenetic biochemical modifications; four are well studied: DNA methylation, genomic imprinting, histone modification, and miRNA. DNA methylation regulates DNA expression and silencing of repeat elements in the genome. Histone modifications are important in transcriptional regulation and often associated with DNA methylation. Genomic imprinting is a parent of origin-specific allele where one parental allele is silenced. Characterized by global hypomethylation and hypermethylation, the aberrant molecular landscape of tumorigenesis consists of both inherited and acquired epigenetic abnormalities. The hypomethylation activates proto-oncogenes and increases genomic instability, while the hypermethylation silences genes. While oncogenes are activated through dominant mutations or overexpression of a gene, tumor suppressor genes become silenced. The epigenetic chemical tags effect chromatin and histone packaging that allows genes to be turned on or turned off. For example, in normal cells, CpG island promoters are generally not methylated and active. Similarly, the chromatin is open in a normally active tumor suppressor gene. During tumorigenesis, tumor suppressor gene CpG island promoters become methylated, resulting in the formation of silent chromatin structures, thus silencing the promoter region. These biochemical tags, or marks on the genetic chromatin, influence whether or not a gene is expressed.
393
FIGURE 11.1. Genetic alterations in cancer. (See color insert.)
394As the global exposure to chemical pollutants, ionizing radiation, and toxic metals is shown to increase cancer incidence, epigenetic cancer research is enhancing our knowledge of how “pathologic epigenetic changes are increasingly considered as alternatives to mutations and chromosomal alterations in disrupting gene function” (Hatziapostolou & Iliopoulos, 2011, p. 1682). Examples of epigenetic chemical modifications that initiate cancer by altering gene (protein) expression are those associated with cigarette smoking and toxic metals. These are included later in this chapter.
Sporadic Mutations
In contrast to inherited cancer syndromes, the term sporadic cancer is used to describe disease that happens de novo, or by chance. Although sporadic cancers are not linked to an identifiable, heritable cancer susceptibility gene, they nevertheless involve genetic and chromosomal alterations. This category comprises 90% to 95% of all cancers not attributable to gene mutations or chromosomal aberrations. Theoretically, a majority of these spontaneous cancers are related to epigenetic biochemical modifications. Cancer-inducing changes can result from spontaneous mutations involving environmental factors such as radiation, viruses, carcinogenic chemicals, toxic metal exposure, or even certain lifestyle factors. Sporadic cancers can occur at an early age of onset; however, for the majority of individuals without heritable risk factors, spontaneous or sporadic cancer risk increases as humans age. This is a direct reflection of cancer’s multistep progression of genetic insults.
GENOMIC MUTATIONS THAT DISRUPT CELL DIFFERENTIATION AND FUNCTION IN CANCER
Genomic mutations can occur in one or a combination of the four major genetic mechanisms described above. There are four types of classic germline and somatic cell genomic mutations that act at the molecular level to disrupt normal cell growth. Table 11.1 provides examples of each type of mutation and describes the genetic nature of each.
DNA Repair Genes
Certain categories of genes have been identified as important players in the development of cancer due to their functions in growth, inhibition, and repair. The protein products of DNA repair genes monitor and correct errors or damage to DNA from a variety of exogenous and endogenous insults that would otherwise have potentially harmful repercussions. When a mutation in this type of gene occurs, the cell loses its ability to repair damaged DNA sequences and cell division results in daughter cells that not only carry the mutation and DNA damage, but are more susceptible to further insult. Impaired DNA repair genes can be inherited as germline mutations, silenced through epigenetic mechanisms, or result from acquired somatic changes. There are a variety of DNA repair mechanisms, such as nucleotide excision repair, base excision repair, and mismatch repair. Overall, dysregulation of DNA repair predisposes a cell to genomic instability, which is a classic characteristic of cancer. An example of impaired DNA repair can be seen in xeroderma pigmentosum, which involves impairments of genes that are normally involved in the repair of ultraviolet-light-induced damage. Ineffective repair results in heightened sensitivity to sunlight and a predisposition for skin cancers.
395TABLE 11.1 Genomic Mutations That Disrupt Cell Differentiation and Function in Cancer | ||
Type of Alteration | Gene Mutations and/or Chromosomal Biomarkers | Cancer Diagnosis |
DNA Repair | MSH2, MSH6, PMS2, MLH1 | Hereditary colon cancer |
XPA, XPC | Xeroderma pigmentosum, skin cancers | |
ATM | Lymphoma, leukemia, breast cancer | |
MRE11 | Breast cancer | |
WRN | Sarcoma, colorectal, skin, thyroid, pancreatic cancers | |
Chromosomal Alterations | Chromosomes 9 and 22 | Chronic myeloid leukemia |
Chromosomes 8 and 14 | Burkitt lymphoma | |
Oncogenes | ERBB2 (HER-2) | Breast cancer |
MYC | Lung cancer | |
RAS | Pancreatic, colon, and lung cancers | |
EGFR | Lung caner | |
MITF | Melanoma | |
Mutant B-Raf | Melanoma | |
Cyclin E | Liver cancer | |
B-Catenin | Colon cancer | |
K-rasv12 | Pancreatic cancer | |
K-rasmut | Pancreatic cancer | |
Cyclin D1 | Esophageal, colon, pancreatic, squamous, nasopharyngeal cancers | |
396Tumor Suppressor Genes | BRCA1, BRCA2 | Breast and ovarian cancer |
RB1 | Retinoblastoma | |
WT1, WT2 | Wilms tumor | |
CDKN2A | Melanoma | |
APC | Colorectal cancer | |
VHL | Kidney cancer | |
NF1, NF2 | Nerve tumors, brain cancer | |
TP53 | Has been identified in over half of human cancers |
Source: GeneReviews (2014).
Oncogenes
For more than 50 years, cancer initiation theories have centered on activation of oncogenes or inactivation of tumor suppressor genes. In humans, there are more than 40 proto-oncogenes, which are a class of genes that contribute to a variety of growth-control pathways, such as growth factors, signaling enzymes, transcription factors, or growth factor receptors. The normal growth-control pathway involves the binding of growth factors to receptor sites on the cell’s membrane, which in turn trigger signaling enzymes to activate transcription factors within the nucleus. The transcription factors subsequently activate specific genes involved in cell growth and division.
There are a number of mechanisms by which proto-oncogenes, vital to the health and function of a cell, become harmful, cancer-promoting oncogenes. Conversion of proto-oncogenes to oncogenes occurs through:
397 Point mutations, deletions, or insertions leading to a hyperactive gene product
Point mutations, deletions, or insertions in the promoter region of a proto-oncogene leading to increased transcription
Gene amplification events leading to extra chromosomal (aneuploidy) copies of a proto-oncogene
Chromosomal translocations can relocate a proto-oncogene to a new chromosomal site, leading to higher-than-normal gene expression
Chromosomal translocations that lead to a fusion between a proto-oncogene and a second gene, producing a fusion protein with oncogenic activity
Oncogene activation can result from either inherited predisposition or more commonly through acquired/environmental triggers, such as exposure to retroviruses or carcinogens. Oncogenes behave in a dominant fashion, which means only one abnormal allele is necessary for expression and subsequent deleterious effects. Whatever the mechanism for oncogene activation, the effect is growth promotion and the potential for cancer. Examples of oncogenes include the HER2 gene implicated in aggressive breast cancer and the MYC gene associated with Burkett lymphoma and lung cancer.
Certain pathogens, such as viruses, can contribute through the insertion of viral RNA into a human cell. Infectious agents, mainly retroviruses, act as oncoviruses that contribute to a variety of malignancies (Table 11.2). Collectively, these account for up to 20% of cancers around the globe. Although bacteria have the ability to become oncogenic, not much is known about specific bacteria. Viruses and bacteria exert their oncogenicity by three common pathways:
Inserting their genome into human DNA
Hijacking the epigenome and tagging genes with biochemicals (miRNAs, DNA methylation, or histone modifications) that inhibit or silence tumor suppressor genes and promote transcription of oncogenes
Infecting human cells and indirectly transforming the cell’s progression toward cancer growth and oncogenic maintenance.
Tumor Suppressor Genes (Anti-Oncogenes)
Tumor suppressor genes represent an innate mechanism of cancer prevention. These genes regulate cell growth by limiting cell division, aiding in DNA repair, and inducing programmed cell death in cells that could otherwise cause harm. Both oncogenes and tumor suppressor genes contribute to cancer development, but a primary difference is the inhibition (or silencing) of tumor suppressor genes—as compared to the expression of oncogenes—that potentiates unregulated growth. When silenced, a tumor suppressor gene loses its ability to repair genetic insults, halt the continuation of damaged cell lines, and maintain growth within healthy boundaries.
Tumor suppressor genes can be silenced through chromosomal alterations such as translocations, inherited gene mutations, or by multiple acquired mutations as a consequence of environment and lifestyle influences. In contrast to oncogenes, tumor suppressor genes are recessive in nature; both alleles of the genes must be abnormal for significant gene malfunction to occur. Since mutations from an inherited etiology already involve one abnormal copy, only one more insult is required to silence the gene. Compare this to cells without a hereditary cancer predisposition, which require two damaging events to knock out the function of the tumor suppressor gene. Well-known examples of tumor suppressor genes implicated in hereditary breast cancers include BRCA1 and BRCA2.
398TABLE 11.2 Oncogenic Pathogens | |
Oncovirus* | Cancer Type |
Human papillomavirus (HPV) | Cervical |
Human polyomavirus (HPMV) | Mesotheliomas, brain tumors |
Epstein–Barr virus (EBV) | Lymphoproliferative diseases and nasopharyngeal |
Kaposi sarcoma herpesvirus | Kaposi sarcoma and primary effusion lymphomas |
Hepatitis B and hepatitis C viruses | Hepatocellular |
Human T-cell leukemia virus-1 (HTLV1) | T-cell leukemias |
Oncopathogen** | Cancer Type |
Helicobacter pylori | Gastric |
Chlamydia trachomatis | Cervical |
Helicobacter | Biliary tract |
Staphylococcus aureus (i.e., chronic osteomyelitis) | Sinus tracts, skin ulcers, squamous cell |
Adapted from *Pagano et al. (2004) and **Chang and Parsonnet (2010).
GENOMICS OF CHILDHOOD CANCERS
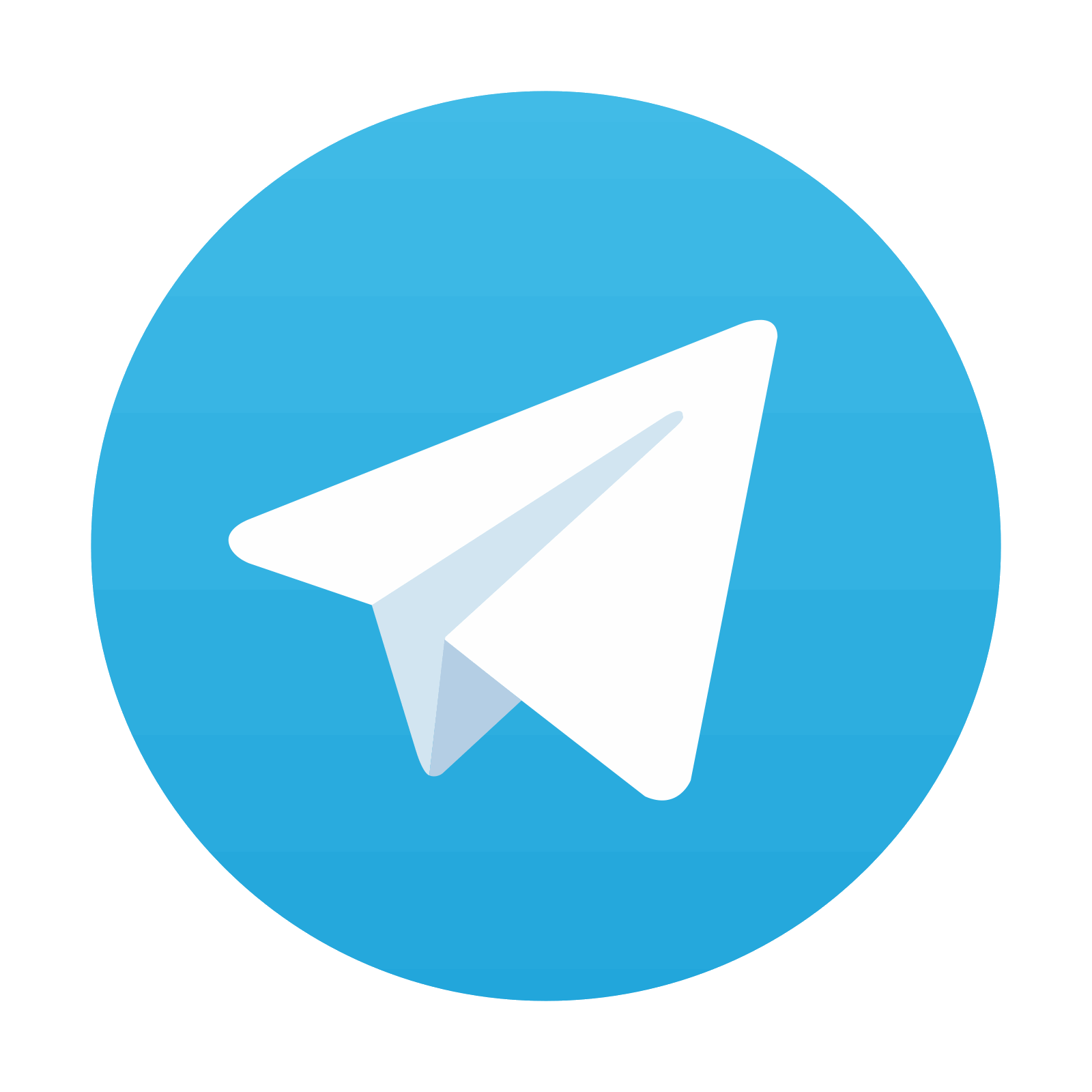
Stay updated, free articles. Join our Telegram channel
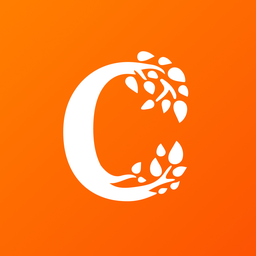
Full access? Get Clinical Tree
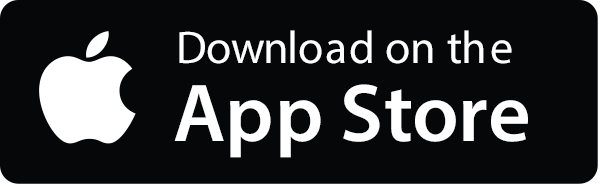
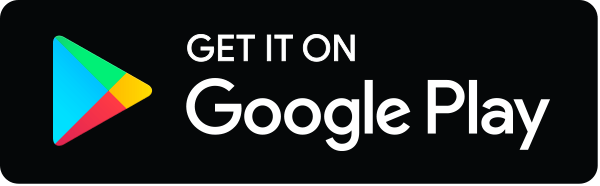