Chapter 30 Supporting the newborn infant
Learning outcomes for this chapter are to:
1. describe the physiological and social development of babies as they make the transition to extrauterine life and in the first weeks after birth
2. develop assessment skills to monitor infant health, wellbeing and responses to physiological changes—neonatal assessments and screening tests, and growth parameters
3. enable midwifery practice that informs, educates and supports the baby’s mother in relation to normal baby behaviour patterns, daily care and the minor complexities of the neonatal period
4. explain the minor complexities of the neonatal period
5. discuss how to assist mothers to identify and manage baby emergencies.
This chapter provides an overview of neonatal physiology and the transition to extrauterine life. It explains the knowledge and skills that midwives need for assessing neonatal wellbeing, including puerperal assessment. It concludes with an overview of the most common minor complications that affect the neonate’s health.
INTRODUCTION
The neonatal (or newborn) period lasts from birth until 28 days of age (London et al 2006). Midwives care for women and their new babies for up to six weeks postpartum. In Australia, the period of postnatal midwifery care varies between states and between urban and remote settings. In New Zealand, women and babies are generally discharged from midwifery care at six weeks postpartum. Initial and ongoing monitoring of the health and wellbeing of the newborn infant is one of the primary purposes of ongoing midwifery care.
Midwives are part of families’ lives for a very short period. One of their major roles is to support and enable women to be confident mothers. Knowledge of her baby’s behaviour and development, understanding how to care for her baby, and reinforcement that she is a capable mother, all engender the mother’s confidence in her ability to mother her baby.
Caring for newborn babies is not always part of young women’s experiences before they have their own children, and therefore midwives are often in the position of supporting and guiding women as they learn to care for their new infant. In these circumstances, it is tempting for professionals to regard the baby as ‘theirs’ and to give women information in an instructional fashion that relegates the baby’s mother to the role of ‘outside’ caregiver, afraid to use her own common sense or to do things for her baby without permission from the ‘expert’. At all times the midwife’s client is the woman. The woman is the baby’s mother; most know whether their baby is doing well or not. Midwifery care is provided in partnership with the baby’s mother, and therefore all midwifery observation, care, treatment and referral are undertaken only in consultation and agreement with her.1
NEONATAL PHYSIOLOGY AND THE TRANSITION TO EXTRAUTERINE LIFE
This section explores neonatal physiology and the transition to neonatal life, in sections related to body systems.
Respiratory system
While functional at birth, the alveoli continue to mature until about eight years of age (London et al 2006; Michaelides 2004a). A term baby has approximately 8% of the adult number of alveoli. Pulmonary, vascular and lymphatic structures differentiate during the first 20 weeks of gestation. Alveolar ducts have begun to develop by 24 weeks of pregnancy and primitive alveoli appear by 28 weeks gestation. There are two types of alveolar cells: type I cells necessary for gas exchange, and type II cells for the synthesis and storage of surfactant (London et al 2006).
Surfactant
Surfactant is produced in the lungs in increasing amounts from 32 weeks gestation. It comprises phospholipids and specialised protein molecules that reduce the surface tension of the alveolar fluid at the air/alveolar interface, and contributes to the elasticity of lung tissue (London et al 2006; Varney et al 2004). As gestation increases, the lining of the alveoli becomes thinner, increasing the surface area for eventual gas exchange and effectively fusing the capillary endothelium and alveolar basement membranes (Michaelides 2004a; Novak 2005a). At term, the alveoli are lined with surfactant. Because surfactant reduces the surface tension in the alveoli, it reduces the tendency of the alveoli to collapse with each breath due to the elastic recoil of the lungs, providing the lung stability for effective gas exchange. Surfactant increases lung compliance and thus reduces the pressure required to inflate the lungs for inspiration (Michaelides 2004a; Novak 2005a; Ricci & Kyle 2009; Varney et al 2004). Synthesis of surfactant is thought to be affected by acidosis and/or hypothermia (Michaelides 2004a).
Fetal breathing movements
Respiration after birth is a continuation of an intrauterine process. From as early as 11 weeks gestation, fetal breathing movements occur in order to develop the chest muscles and diaphragm (London et al 2006). As the fetus grows, the frequency and strength of breathing movements increases, until they reach 30–70 breath movements per minute and are present 40%–80% of the time (Davis & Bureau 1987, cited in Michaelides 2004a, p 530).
Lung fluid
Lung fluid is not surfactant. It is a clear fluid that facilitates cell proliferation and differentiation. Prior to labour, production of lung fluid is reduced. At birth the lungs’ primary function changes from secretion of fluid to the absorption of gases. It is possible that the catecholamine surge at birth is the final trigger for this change (Ricci & Kyle 2009). Lung perfusion, commenced in late labour and continued after birth, facilitates the absorption of lung fluid from the alveoli by osmosis (Mercer & Skovgaard 2002, cited in Davies & Richards 2008). At birth, the remaining lung fluid (approx 80–100 mL) is either expelled through the upper airways or absorbed through temporarily more permeable alveolar epithelium into the interstitial tissue (Michaelides 2004a; Ricci & Kyle 2009). Within two hours of the birth of a healthy term neonate, about 80% of the fluid has been reabsorbed and is completely absorbed from the alveoli within 12–24 hours. The fluid is absorbed from the interstitial tissue into the pulmonary vasculature and lymphatic drainage over the next few days (Novak 2005a; Ricci & Kyle 2009).
Clinical point
Monitoring ‘wet’ lungs
Occasionally audible rales and/or rhonchi may be present at birth. These sounds may be associated with lung fluid that has not yet been reabsorbed.
• If the sounds are diminishing in audibility and harshness at this time: continue to check every 15 minutes.
• If the baby remains well and the sounds continue to diminish: continue to monitor until they have gone. They should have substantially cleared within an hour of birth.
• Refer to a medical practitioner if there are any or all of:
Note: Transient tachypnoea of the newborn can occur in otherwise healthy babies (see Ch 39).
Research findings
Oropharyngeal suctioning at birth
Routinely suctioning lung fluid from the baby’s nose and oropharynx at birth was abandoned in most places more than a decade ago. However, where meconium is present in the liquor amnii, suctioning is practised, especially before the baby has taken the first breath.
Recent research has shown that even in the presence of meconium, routine suctioning of a healthy newborn’s oropharynx and nasal passages prior to the birth of the baby is contraindicated and may even be harmful (Vain et al 2004) (see Ch 37).
First active breath
The respiratory and cardiovascular changes that occur immediately after birth happen simultaneously and are mutually dependent (Davies & Richards 2008). while much is known about the initiation of respiration, the precise mechanism that triggers the first actine breath is not get fully understood. Research continues to identify the precise process involved (Rieci & Kyle 2009). Most babies take their first extrauterine breath at the time of birth.
• uterine contractions in the second stage of labour may result in enhanced perfusion that begins to fill the fetal pulmonary capillary bed (Mercer & Skovgaard 2002, cited in Davies and Richards 2008, p 110)
• compression of the chest wall during birth and the recoil of the chest wall immediately after birth. Chest compression and recoil expand intrathoracic capacity, stimulating the baby to take a breath. Lung fluid is displaced through the nose and mouth or across the alveolar walls into the interstitial space, and the mechanism essential for effective respiration and pulmonary gas exchange is triggered (Novak 2005a). Significant negative intrathoracic pressure of up to 9.8 kPa (100 cm of water) is produced as the lung fluid is emptied and the pulmonary tissue and vasculature expand. Much less pressure is needed for subsequent breaths (Farrell & Sittlington 2003a).
• chemoreceptor stimulation by reduction in oxygen and increase in carbon dioxide in the blood. It is possible that normal labour causes mild hypoxia, hypercapnia and acidosis, which play a role in stimulating neonatal respiration. Physiological hypoxia and hypercapnia trigger the establishment of the respiratory drive in the respiratory centre in the medulla oblongata
(Farrell & Sittlington 2003a; Novak 2005a). However, Blackburn (2007) states that chemoreceptors in the neonate are less responsive in the first few days after birth and emphasises the major role of chest recoil and absorption of fluid in facilitating the first breaths. Davies and Richards (2008), in contrast, describe Mercer & Skovgaard’s 2002 model which postulates that increased perfusion and oxygenation from second-stage contractions and delayed cord clamping are the primary factors in the initiation of respiration.
• other external stimuli such as cold, light noise and touch are secondary triggers for the baby to take the first breath.
Neonatal breathing patterns
At birth, the respiratory system is immature. Neonates have shallow, irregular breathing and breathe through the nose for the first two to three months. ‘Babies are obligatory nose breathers and do not convert automatically to mouth breathing when nasal obstruction occurs’ (Farrell & Sittlington 2003a, p 729). On auscultation, breathing may sound noisy and wet during the first one to two hours while any remaining fluid is cleared from the lungs (Varney et al 2004) (see Clinical point on wet lungs, previous page).
The normal baby has an average respiratory rate of 40 breaths/minute (range is 30–60 breaths/minute; see Table 30.1), although the rate may be elevated in the first two hours after birth (Davies & Richards 2008; London et al 2006). Breathing is diaphragmatic—the chest and abdomen rise and fall together. Such symmetry confirms that the lungs and diaphragm are functioning correctly. Newborn babies commonly have periods of irregular breathing, particularly during random-eye-movement (REM) sleep. The irregular pauses between breaths usually last 5–15 seconds. These pauses are not associated with changes to the baby’s colour or heart rate. Pauses of less than 20 seconds are not usually of clinical concern (London et al 2006; Ricci & Kyle 2009).
Table 30.1 Neonatal breathing patterns
Normal variations | Outside normal variations (any or all of these) | |
---|---|---|
Rate | More than 60 breaths/minute (sleeping or in quiet alert state) | |
Rhythm | ||
Chest/abdominal movement | Synchronised diaphragm and abdominal movement | Subcostal and/or intercostal in-drawing |
Nose | Breathes through the nose, no nasal flaring | Flaring of nostrils |
Sound | Silent | Expiratory ‘grunt’ |
Colour | Pink | Pale/cyanosis |
(See Ch 39 for respiratory distress syndrome.)
While an elevated respiratory rate is a sign that the baby is unwell, tachypnoea cannot be assessed without taking the baby’s other behaviours into account, such as recent crying, feeding or sleeping (Farrell & Sittlington 2003a; Michaelides 2004a). A baby’s cry is loud and of medium pitch. Over time, mothers learn to distinguish variations in crying and to attach meaning to them (e.g. hungry, wet, in pain).
Cardiovascular system
The fetal circulation is completely different to that of postnatal circulation (see Fig 30.2) and is discussed in detail in chapters 20. Blood oxygenated in the placenta reaches the fetus via the umbilical vein. About 50% perfuses the liver, and the remainder enters the inferior vena cava by way of the ductus venosus. This blood mixes with the venous return from the liver and the lower half of the fetal body, and then flows to the right atrium via the inferior vena cava. Streaming of the blood through
Clinical point
the right atrium causes it to cross to the left atrium via the foramen ovale. This blood is more highly oxygenated than blood returning by the superior vena cava, and constitutes the left ventricular output supplying the first part of the aorta. Thus the coronary and carotid arteries receive the most oxygenated blood available. Blood returning to the right atrium through the superior vena cava flows into the right ventricle. Only a small proportion of the right ventricular blood enters the pulmonary circulation; most passes through the ductus arteriosus into the arch of the aorta. The remainder flows to the lower parts of the fetal body. Changes from fetal to post-birth circulation are dealt with in more detail in the following sections.
Post-birth respiratory and cardiovascular changes occur simultaneously and are mutually dependent (Davies & Richards 2008; Farrell & Sittlington 2003a).
Cardiovascular adaptations at birth
Cardiovascular adaptations that occur at birth are outlined in Table 30.2. The temporary fetal structures that support the fetal circulation are reversed after birth. These structures are the foramen ovale, ductus arteriosus, ductus venosus and the hypogastric arteries. The increase in catecholamine release encouraged by birth stimulates increased cardiac contractility and output (Ricci & Kyle 2009).
Table 30.2 Fetal and neonatal circulation
System | Fetal | Neonatal |
---|---|---|
Pulmonary blood vessels | Constricted, with very little blood flow; lungs not expanded | Vasodilation and increased blood flow; lungs expanded; increased oxygen stimulates vasodilation |
Systemic blood vessels | Dilated, with low resistance; blood mostly in placenta | Arterial pressure rises due to loss of placenta; increased systemic blood volume and resistance |
Ductus arteriosus | Large, with no tone; blood flow from pulmonary artery to aorta | Reversal of blood flow; now from aorta to pulmonary artery because of increased left atrial pressure. Ductus is sensitive to increased oxygen and body chemicals and begins to constrict. |
Foramen ovale | Patent, with increased blood flow from right atrium to left atrium | Increased pressure in left atrium attempts to reverse blood flow and shuts one-way valve |
Pulmonary oxygenation of blood
In uterine life, 5%–10% of cardiac output enters the pulmonary circulation in order to meet pulmonary cellular growth and nutrition needs. High pulmonary vascular resistance and the patent ductus arteriosus ensure that the remainder of the cardiac output enters the arterial system. At birth, adjustments must be made to the baby’s circulation so that deoxygenated blood flows to the lungs for oxygenation. This involves several mechanisms. The expansion of the lungs and associated lowered pulmonary vascular resistance which occurs with the first breath enables virtually the entire heart’s output to enter the pulmonary circulation. Oxygenated blood returning to the heart via the pulmonary veins increases the pressure within the left atrium (London et al 2006; Michaelides 2004a). This triggers the closure of the foramen ovale.
Closure of the foramen ovale
The foramen ovale is an opening in the atrial septum created by a flap of tissue that is kept open in fetal life by the higher pressure of the blood in the right atrium. At birth, the vessels in the umbilical cord constrict, irrespective of whether the cord is clamped early or late, and the pulmonary vasculature expands. These events result in a fall in right atrial pressure and a concomitant rise in left atrial pressure. The pressure alterations, especially those in the left atrium, cause the flap of the foramen ovale to be pressed flat against the septum and therefore to functionally close. Flow murmurs in the first 24–48 hours postpartum may reflect incomplete closure of the foramen ovale and may persist until the pressures stabilise so that the foramen stays closed. In the first days after birth, closure is reversible; for instance if pulmonary vascular resistance is high—for example, when crying—transient cyanotic episodes may occur if the foramen temporarily reopens. The foramen anatomically closes within the first year of life (Farrell & Sittlington 2003a; London et al 2006; Novak 2005a).
Closure of the ductus arteriosus
In fetal life, the ductus arteriosus is nearly as wide as the aorta. Blood is shunted directly from the pulmonary artery to the aorta via the ductus
arteriosus, thereby avoiding the lungs. At birth, the pulmonary vascular resistance falls and the amount of blood being shunted to the aorta via the ductus arteriosus is substantially decreased. Constriction of the muscular walls of the ductus arteriosus occurs almost immediately after birth. Decreasing levels of maternal prostaglandins (previously supplied by the placenta) and rising arterial oxygen tension trigger vasoconstriction. The ductus arteriosus is usually functionally closed by 8–10 hours after birth, but will not anatomically close for several more months. Indeed, before pressures stabilise, a temporary reverse shunt through the ductus arteriosus may persist for a few hours. Moreover in some babies the ductus has been shown to be intermittently patent up to three days after birth (Blackburn 2007; Farrell & Sittlington 2003a; Michaelides 2004a; Novak 2005a).
Adaptation of other temporary fetal cardiovascular structures
Clamping the umbilical cord causes the ductus venosus and the hypogastric arteries to functionally close almost immediately. Anatomical closure occurs within two to three months of birth. The resulting fibrous structures are known as the ligamentum venosum and ligamentum teres. The superior vesical arteries are the vestiges of the hypogastric arteries (Farrell & Sittlington 2003a).
Characteristics of neonatal cardiac function
• Heart rate—in the first week after birth the average heart rate is 110–150 beats per minute in a quiet, healthy term neonate. However, wide fluctuations occur deplending on whether the baby is active, feeding or asleep (Farrell & Sittlington 2003a; London et al 2006).
• Peripheral circulation—the healthy term baby’s hands and feet are mildly cyanosed (acrocyanosis) for several hours after birth until peripheral perfusion is complete.
• Blood volume and blood pressure—systolic blood pressure varies with gestation, birthweight and neonatal activity such as crying. It is not usually assessed in healthy term infants (London et al 2006). At birth, the total circulating blood volume is 80 mL/kg of bodyweight (i.e. 280 mL for a 3.5 kg baby) (Blackburn 2007). The relatively low total circulating blood volume means that quite small quantities of blood represent a large blood loss for the neonate (e.g. 10% of adult blood volume is 800 mL; 10% of a 3.5 kg baby’s blood volume is 28 mL). Attentive concern is required when assessing blood loss from the umbilical cord or the amount of blood taken for laboratory analysis.
Heart murmurs
Heart murmurs are sounds that may be heard as: blood flow is disturbed as it crosses an abnormal valve; as it flows under pressure through a defect in either the atrial or ventricular septum; or sometimes when there is increased flow as it crosses a normal valve. The murmur reflects turbulent blood flow, typically from the pressure difference between adjacent cardiac structures.
Cardiac murmurs in the neonate may be normal (physiological, benign, flow, transitional, etc.) or abnormal (pathological). Most neonates (90%) will have ‘soft’ murmurs which are transient and not associated with anomalies (London et al 2006). Murmurs can be classified according to their:
• timing (systolic, diastolic, continuous)
• intensity (1–6 systolic, 1–4 diastolic)
• pitch (high or low frequency)
Clinical point
Neonatal heart murmurs
Murmurs are not heard if there is nothing to make the flow turbulent. Many serious cardiac malformations are silent, and therefore midwives need to always be observant for systemic signs of cardiac compromise—colour, respiratory rate, muscle tone, feeding problems, and so on (see Ch 39).
Clinical point
Why large cardiac septal defects are usually silent
Flowing fluid does not make a noise if it is not under a lot of pressure. Think of the absence of noise when water flows through a wide-bore hose. When fluid is forced through a small opening, the pressure is raised and the resulting turbulence creates a noise. Think of the way water begins to hiss when pressure is increased by a thumb partially closing the opening of a wide-bore hose. Large defects in the atrial or ventricular septa allow the blood to flow through the defect with little or no change in pressure. Consequently there is no turbulence and no sound is made. Diagnosis of large cardiac anomalies is nearly always made through clinical observation of babies and their behaviour.
Temperature regulation
Newborn babies sustain life within a narrow range of core (internal) body temperatures, generally 36.5–37.5oC (Blackburn 2007). The body uses a number of mechanisms to balance heat loss and heat production in order to maintain the core temperature within this range despite a wide range of environmental temperatures. Balancing heat loss and gain is known as thermoregulation. The range of ambient temperatures that maintains a baby’s core body temperature using minimum oxygen consumption at a minimum metabolic rate is called the thermoneutral range (Blackburn 2007; London et al 2006; Ricci & Kyle 2009).
At birth, babies’ thermoregulatory mechanisms are not fully developed, although these become progressively more efficient. Limited response to temperature changes in the first 24 hours of life results in babies being particularly susceptible to chilling during this time. By 24–48 hours of age, healthy term neonates are able to increase their heat production up to 2.5 times in response to cold; however, they remain susceptible to environmental temperature changes. Until the mechanisms controlling thermoregulation (and heat loss in particular) stabilise, the thermoneutral range of babies is at a higher environmental temperature than older children and adults. During the first weeks of life, warmed rooms, or extra clothing and blankets if babies are taken outside, are often required (Blackburn 2007; London et al 2006; Michaelides 2004b).
Neonatal features contributing to heat loss
• Large surface-area to mass ratio (approximately three times greater than adults). In particular, a baby’s head (the major heat-loss area in a clothed baby), comprises a larger proportion of the body than that of an adult.
• Blood vessels closer to the skin, so changes in the ambient temperature more readily influence the circulating blood, thereby influencing the temperature-regulating centre in the hypothalamus.
Neonatal heat loss
Heat is very easily lost at birth, when autonomic thermoregulation is at its least efficient and the baby is wet. Hypothermia (a temperature less than 36.5°C) can occur rapidly unless active steps are taken to prevent heat loss.
There are four ways in which babies can lose heat from their body surface (Blackburn 2007; London et al 2006; Michaelides 2004b; Novak 2005b):
• Evaporation—heat loss occurs when water evaporates as vapour from the skin. Babies are particularly vulnerable to cooling by evaporation at birth and during bathing if the environmental temperature is too low. A birthing-room temperature of at least 25–28°C is needed, to prevent heat loss at birth. Minimising the time the baby is wet by careful drying also helps to prevent heat loss by evaporation.
• Convection—cooler air passing over a warm baby causes heat loss by convection. Preventive measures include eliminating cool air flows from draughts and air conditioning. Oxygen given by mask or by oxygen catheter can cause heat loss by convection.
• Conduction—coming into direct contact with a colder surface can rapidly cool a baby. Cold hands, clothes and bedding, weighing-scale trays and stethoscope diaphragms all belong in this category. These items should be warmed before coming into contact with the baby’s skin.
• Radiation—radiant heat loss happens when heat is transferred from the body to cooler objects not in direct contact with the baby; for example, bassinets or cots placed against external room walls or under windows can cause heat loss by radiation.
Clinical point
Heat loss in preterm and SGA babies
Preterm infants and small-for-gestational-age (SGA) babies have less adipose tissue and a greater surface-area to mass ratio than full-term babies. Furthermore, the lower the gestational age, the less brown adipose tissue is deposited and the less the body and limbs are flexed. Consequently these babies are extremely vulnerable to hypothermia. They require a higher environmental temperature to maintain a thermoneutral range (London et al 2006).
Neonatal thermogenesis
Neonates rarely shiver. They generate heat by increasing their metabolic rate using muscular activity such as moving limbs and suckling, and by chemical thermogenesis (heat production), also called non-shivering thermogenesis (Blackburn 2007).
Thermogenesis and brown adipose tissue
Heat production by chemical means involves the metabolism of brown adipose tissue (BAT). Brown fat is of particular importance in human neonates because it has the ability to dissipate stored energy as heat. In contrast to other cells, including white adipocytes, brown adipocytes express mitochondrial uncoupling protein 1 (UCP1), which gives the cells’ mitochondria an ability to uncouple oxidative phosphorylation and utilise substrates to generate heat, rather than via adenosine-5’-triphosphate (ATP). Exposure to cold leads to sympathetic stimulation of brown adipocyte via norepinephrine binding to beta-adrenergic receptors.
Clinical point
The heat produced in brown fat can be imaged using a thermal (infrared) camera. Images of the skin of an unwrapped baby sleeping at room temperature show ‘hot spots’ which reveal overlying brown fat deposits in the neck and the interscapular area.
At term, BAT is deposited around the nape of the neck and mid-scapular area, under the clavicles and in the axillae, around the kidneys, adrenal glands and large vessels in the neck and in the mediastinum (London et al 2006; Novak 2005b). Maternal prostaglandins and adenosine prevent non-shivering thermogenesis in utero. BAT is only activated after birth. Brown fat stores are not renewable once they have been used (Michaelides 2004b).
Brown adipose tissue is so-named because of the colour that results from the tissue containing a more extensive blood supply and greater numbers of intracellular organelles such as mitochondria than in white adipose tissue. Neonatal cooling causes noradrenaline, other catecholamines and thyroid hormones to be released so that rapid lipolysis of BAT and consequent heat production is induced. Oxygen and glucose are required in the metabolism of brown fat. Brown fat lipolysis uses up to three times more oxygen than other tissues. Normal oxygen consumption, conservation of brown fat stores and maintenance of core body temperature are promoted by keeping babies in a thermoneutral range (Blackburn 2007; Farrell & Sittlington 2003a; London et al 2006; Novak 2005b).
Maintaining temperature after birth
At birth, healthy term babies are placed against their mother’s body skin-to-skin, dried and covered with a warm blanket to maintain their temperature. A mother’s body conducts direct warmth to the baby, and the blanket traps a layer of warm, insulating air around the baby (Blackburn 2007; Michaelides 2004b). Studies of preterm babies in Colombia and England showed that skin-to-skin contact between the mother’s breasts satisfactorily maintains body heat (Sleath 1985; Whitelaw et al 1988). More recent studies have suggested that the maternal body temperature fluctuates in response to the baby’s temperature so that a stable temperature is maintained. Furthermore, skin-to-skin contact appears to stabilise and support cardiorespiratory function (Davies & Richards 2008).
The following methods are commonly used to conserve heat in newborn infants (McHugh 2004).
• Placing the baby in immediate skin-to-skin contact with the mother.
• Drying the baby thoroughly immediately after birth (this also encourages the onset of respiration).
• Encouraging the mother to initiate skin-to-skin contact on an ongoing basis.
• Pre-warming any hats, blankets and clothing prior to the birth.
• Pre-warming the resuscitation area.
• Maintaining the temperature in the birthing room at >25°C.
• Replacing wet blankets or towels after drying the baby.
• Not bathing the baby until temperature has been stable for at least two hours.
• Not placing the bassinet against windows, outside walls or doorways.
• In cooler environments, keeping the baby’s head covered and body well wrapped.
Wrapping babies
While warm towels, blankets and clothing are a necessity and many babies appear to prefer being firmly wrapped, overly tight wrapping prevents the movement that generates heat. The baby’s clothes should not be too tight. It is better to use several thin layers than one or two thick layers (natural fabrics ‘breathe’ better than synthetics). Hats, especially for small and preterm infants, have proved effective in reducing heat loss from the head in cool environments or when heat loss is a concern. When transporting small or sick babies, skin-to-skin contact with the mother or a thermoblanket that retains body heat may be used, depending on the condition of the baby (London et al 2006; Michaelides 2004b).
Hot water bottles and electric blankets
These items are used only for heating fabrics and must never be left in the cot with the baby. This is also true of wheat bags or anything else that retains heat in a similar way, such as gel packs.
Bathing and temperature maintenance
Babies are no longer bathed immediately after birth unless there is a risk of vertical transmission of an infection from the mother, such as hepatitis B or HIV. Bathing soon after birth increases the risk of hypothermia, and therefore it should not be undertaken until the baby’s temperature has stabilised (Leveno et al 2003). Breastfeeding is more important, both to initiate feeding and provide fuel for the baby’s metabolic processes, including temperature maintenance, and to enhance the mother–baby relationship. Skin-to-skin contact seems to influence neurobehavioural responses so that rooting and latching are easier (Ferber & Makhoul 2004).
Although babies’ thermoregulatory mechanisms become more efficient as the days pass, bathing remains a time when they can be easily chilled. The following precautions should always be taken (London et al 2006; Michaelides 2004b; Varney et al 2004).
• Bathe the baby in a draught-free room.
• Ensure the ambient temperature is 25–28°C.
• The baby’s temperature should be within the normal range.
• Water temperature should be 36.7°C (similar temperature to skin when tested with the inside surface of an adult’s wrist).
• Warm the towels and clothing.
• Do not expose the baby unnecessarily (e.g. keep wrapped in a towel while bathing the head).
• Dry the baby promptly and thoroughly, especially the hair and in the folds of the skin.
Clinical point
Neonatal temperature measurement
• Axillary temperature—This is the preferred site.
Note: In a hypothermic baby, the reading will be higher than the core as the area contains large areas of brown fat (Bliss-Holtz 1991). As this may make recognition of hypothermia more difficult, behavioural and other physiological signs should also be assessed when the temperature is taken.
• Rectal temperature—It is considered preferable to routinely measure the temperature via the axilla; however, core temperature may be required in individual situations. The rectal temperature measures the core temperature and is the most accurate. It is used when the axillary temperature is abnormal; e.g. check rectal temperature if axillary temperature is <36.5°C.
1 Measuring a newborn’s rectal temperature: The well-lubricated thermometer should be gently inserted no more than 3 cm into the rectum of the term baby and no more than 2 cm in the preterm baby (Blackburn 2007; Fleming et al 1983). The baby’s legs need to be held still during this procedure and great care taken to ensure that the rectum is not damaged. For this reason, many institutions prohibit this procedure.
• Tympanic temperature—As the reading is often affected by environmental temperature, this site is less accurate for newborn babies and should not normally be used. Wells et al (1995) showed that readings were 0.5–1.0°C higher than the core temperature when the environmental temperature was >30°C, and 0.5–1.0°C lower when the environmental temperature was <30°C.
• Skin sites—For a rapid general assessment of temperature, skin sites can be useful.
• Commercial ‘spots’ or ‘tapes’—Used on the skin, these are a non-invasive method for taking the temperature that parents may find useful as the baby grows older.
Impaired thermoregulatory control
Neonatal hypothermia
If the neonate’s temperature falls below 36.5°C, the baby is at risk of hypothermia. Prevention is the best treatment. A mildly hypothermic baby (36–36.5°C) can generally be warmed without difficulty by nestling the baby against the mother skin-to-skin (and covering with a blanket) in a warm (>25°C) room if the neonate’s other assessments are stable, or by placing in an incubator set at 35–36°C. In the latter situation the baby should be clothed but not under a blanket (Blackburn 2007; London et al 2006; Michaelides 2004b; Novak 2005b).
Untreated hypothermia can result in generalised vasoconstriction that includes the pulmonary vessels. Pulmonary vasoconstriction results in decreased pulmonary perfusion that affects pH, PaO2 and PaCO2 levels. Increased PaCO2, and decreased PaO2 and pH levels result in respiratory acidosis. Consequently the baby will exhibit signs of respiratory distress (Farrell & Sittlington 2008).
Clinical point
Relationship between hypothermia, hypoxia and hypoglycaemia in neonates
• Healthy neonates have limited stores of glycogen and brown fat and are vulnerable to rapid heat loss.
• Preterm infants have less glycogen stores and brown fat and greater vulnerability to heat loss than term babies.
• Hypothermia leads to brown fat lipolysis to restore the temperature. The process consumes oxygen and glycogen.
• Hypoglycaemia leads to mobilisation of glycogen stores to restore serum glucose levels. The process of converting glycogen to glucose requires oxygen and heat. Glycogen stores are depleted.
• Hypoxia leads to anaerobic methods of oxygen production. These inefficient processes require heat and glucose. Glycogen stores are depleted.
Clinical alert!
Clothed babies who become hypothermic in an appropriately warm environment need very prompt medical assessment and investigation, especially for infection. In these circumstances the low temperature is likely to indicate a serious illness (usually an infection) rather than an environmental cause for the hypothermia.
A low (or raised) temperature may be the only sign of early onset Group B streptococcal infection. Unexpected hypothermia in an otherwise well baby is always abnormal.
Neonatal hyperthermia
Hyperthermia is a temperature above 37.5°C and is much less common than neonatal cooling. It can be as harmful as hypothermia if it is ignored. The most common cause of neonatal hyperthermia is underestimation of the effect of the environmental temperature on the baby (e.g. putting the bassinet next to a heater). Babies can develop hyperthermia in response to an infection, environmental overheating or occasionally an underlying medical condition. Recent research has reported a possible association between sudden unexpected death in infancy (SUDI) and the presence of Staphylococcus aureus (Blackwell 2008; Weber et al 2008). Blackwell’s study notes that hyperthermia is one of the symptoms associated with the presence of staphylococcal endotoxins.
Medical consultation is warranted when the baby’s temperature is above 37.5°C without an obvious environmental cause, such as direct sunlight in a well-heated room, or when the baby has a lower temperature but is exhibiting other signs of unwellness, such as not feeding. Similar consultation would be made if the temperature has not returned to normal within an hour of correcting an environmental cause (Farrell & Sittlington 2003a; Michaelides 2004b).
In the case of overheating due to an environmental cause, the baby should be slowly cooled by removing bootees and hat, exposing hands to the air and leaving the baby under a single blanket or sheet. Extremes such as removing all covers or cool bathing must be avoided, as rapid cooling can precipitate excessive heat loss and shock (Michaelides 2004b).
Haemopoietic system
Haemoglobin concentration
The fetal circulation enables fetal blood to be oxygenated at the placental site and to be transported to the brain and other vital organs as efficiently as possible. This is assisted by high concentrations of fetal haemoglobin (HbF), a type of haemoglobin that has a higher affinity for oxygen than adult haemoglobin. After birth the neonate is exposed to higher oxygen levels associated with breathing air. As the red cells with fetal haemoglobin are haemolysed, they are replaced by cells containing adult haemoglobin with a lower affinity for oxygen. Neonatal red blood cells have a shorter lifespan than adult cells, and as they are destroyed this produces elevated serum bilirubin levels and physiological jaundice in some babies (Johnston et al 2003).
The haemoglobin level is initially high (130–200 g/L), and 50%–85% of this is fetal haemoglobin. The levels decrease gradually, with changes apparent in the first week after birth, and fall to approximately 120 g/L by three months of age. Conversion from fetal to adult haemoglobin, which is commenced in utero, is completed in the first one to two years of life (Blackburn 2007; Michaelides 2004a).
Red blood cells, haematocrit and leucocytes
After birth the red blood cell (RBC) count gradually decreases as adult-sized red cells replace the larger HbF red cells. Neonatal RBCs have a lifespan of 80–100 days, approximately two-thirds of the lifespan of adult RBCs. In the first days after birth, the haematocrit (PCV) may increase above fetal levels as a result of placental transfusion and diminished extracellular fluid volume. The RBC count (5–7 × 1012 /L) and haematocrit levels (55%) decrease gradually during the first two to three months of life. During this time, erythropoiesis is suppressed due to the relatively high hyperoxide environment that the neonate is exposed to after birth. Erythropoietin release is reduced due to lack of hypoxic stimulus (Blackburn 2007).
The leucocyte or white blood cell (WBC) count is initially high (1–25 × 109/L) but decreases rapidly. Immature leucocytes, such as band neutrophils, are present in cord blood but disappear as transitional changes take place (Blackburn 2007 usually).
An elevated level of immature neutrophils is usually an indicator of infection.
Clotting factors
Colonisation of the intestine by the bacteria that synthesise vitamin K does not occur until milk feeding is established. Therefore, blood clotting is impaired in the first week postpartum while levels of vitamin-K-dependent clotting factors II (prothrombin), VII, IX and X are low. Adult levels of platelets are present, but their capacity for adhesion and aggregation is reduced (Blackburn 2007; Michaelides 2004a).
Vitamin K prophylaxis
It is unclear why newborn infants have reduced levels of vitamin-K-dependent clotting factors; it has been postulated that this may be related to fetal growth regulation (Blackburn 2007). If vitamin K is not administered parenterally, the natural course is for the vitamin K levels to initially decrease as maternally acquired vitamin K is used. Neonatal vitamin K stores then increases gradually over the first month (Blackburn 2007).
Vitamin K deficiency bleeding
In some babies, the decline in vitamin K after birth leads to vitamin K deficiency bleeding (VKDB). VKDB occurs in an early (<48 hours), classic (2–7 days) or late (1 week to 6 months) form (Blackburn 2007). The natural prevalence of the classic form is 4–17 per 1000 (Zipursky 1999). The risk of late VKDB without and with vitamin K prophylaxis in Europe and Australia is shown in Table 30.3.
Risk expresed as rate per 100, 000 babies | ||
---|---|---|
Australia | Europe | |
No vitamin K | 33.4 | – |
1 dose oral Konakion® | 20 | – |
2 doses oral Konakion® | 4.1 | 2.6 |
IM Konakion® at birth | 0.2 | 0 |
(Source: NZCON 2001)
Breastfed babies are more at risk of haemorrhagic disease than formula-fed infants because formula contains vitamin K. Unfortunately, it is difficult to predict which babies will develop VKDB, as babies without specific risk factors can develop classic VKDB (Zipursky 1999). Because of these factors, prophylactic vitamin K at birth is recommended to prevent VKDB (Blackburn 2007; NZCOM 2001).
Routine vitamin K prophylaxis
The need for routine prophylaxis for all babies has been questioned since Golding et al (1990, 1992) published the results of a study that linked administration of intramuscular (IM) vitamin K to increased risk of childhood cancer. Several later reports have not demonstrated the same relationship (McKinney et al 1998; Olsen et al 1994; Passmore et al 1998; Zipursky 1996). While some methodological criticisms have been made of some of these later studies, in places where routine use of IM vitamin K was stopped or replaced by oral doses subsequent to the Golding et al study, the incidence of VKDB increased (Passmore et al 1998; Zipursky 1996). It is probable that vitamin K prophylaxis is not needed at birth by every baby; nevertheless, because it is not possible to identify those who do need vitamin K with any certainty, prophylaxis is policy in most maternity services. The New Zealand College of Midwives Consensus Statement (2001) supports vitamin K prophylaxis. In Australia, most babies are given vitamin K prophylaxis (NHMRC 2000). A selective policy of vitamin K administration after screening is not appropriate, because without prophylaxis a relatively high incidence of late VKDB occurs.
Informed consent
Depending on local policy, IM or oral vitamin K is given at birth. Maternity care providers have a responsibility to provide access to information and discussion. Parents need time to consider the information related to vitamin K prophylaxis. It is preferable that they receive written information and an opportunity to discuss their choices during pregnancy so that their decision can be recorded in their plan of care.
Dosage
After the Golding et al report, several studies were carried out comparing different dosage regimens (Cornelissen et al 1992; Greer et al 1998). The conclusions drawn from these studies and other studies are as follows:
• IM or oral vitamin K improves the neonate’s coagulation status in the first week (Farrell & Sittlington 2003a).
• A single dose (1 mg) of IM vitamin K at birth effectively prevents classic VKDB (Medsafe 2006).
• The effectiveness of IM vitamin K in the prevention of late VKDB has been established (Loughnan et al 1999, cited in NZCOM 2001). A Cochrane review (2000) compared IM with oral routes of administration of vitamin K (Puckett & Offringa 2000). Evaluation of 11 randomised controlled trials (RCTs) identified that if administered soon after birth, a single dose of IM vitamin K, compared with a single oral dose, provided higher plasma levels of vitamin K in the first few weeks of life when the risk of VKDB is highest. However, multiple doses of oral vitamin K resulted in higher plasma levels at two weeks and two months than a single IM dose.
• Local policies relating to the administration of oral vitamin K may differ. The three-dose regimen is common. The doses are usually spread across the first six weeks of life (e.g. at birth, one week and six weeks) (Ministry of Health 2004).
Immunological adaptations
Functional immaturity of the immune system and lack of exposure to common microorganisms at birth means that babies are very vulnerable to infections, particularly respiratory and gastrointestinal infections. Antibody-mediated and cell-mediated immunity, the inflammatory response and complement factors are different in newborn infants. Neonates cannot localise infections very well, and so an infection is more likely to become systemic. The immaturity of the gut defence mechanism increases the likelihood of both gastrointestinal infection and later development of allergies (Blackburn 2007; Novak 2005b; Varney et al 2004). Both commensal and pathogenic microorganisms begin to colonise the baby during birth. Colonisation occurs first from the maternal genital tract, then from the mother’s skin, and finally from other people and the general environment (Novak 2005b).
Specific immune responses
Antibody-mediated immunity
At birth the neonate has 55%–80% of the adult total immunoglobulin values (IgG, IgA and IgM) (Blackburn 2007).
• Immunoglobulin G (IgG)—the baby’s levels of IgG are equal to or slightly higher than those of the mother, as maternal IgG is transferred across the placenta. Transfer increases progressively until term. The maternal IgG gives the baby some degree of passive immunity to the diseases to which the mother has antibodies, for approximately six months. After birth, levels fall gradually as the transferred IgG is used. The baby does not develop significant production of IgG until after six months of age. Adult levels are reached over four to six years (Blackburn 2007; Michaelides 2004a; Novak 2005b).
• Immunoglobulin A (IgA)—newborn levels of IgA and IgM are low, as the molecules are too large to cross the placenta. IgA is important for localised immunity in the gastrointestinal and respiratory tracts. It is found in saliva, tears, and intestinal mucosa by two to three weeks of age, although adult levels are not reached in the saliva for two months. Maternal secretory IgA is also transferred to the baby in colostrum and breast milk, affording local protection to the gut. IgA levels reach 20% of adult levels by one year (Blackburn 2007; Novak 2005a).
• Immunoglobulin M (IgM)—at term the baby’s IgM level is 20% of the adult value, which is not reached for two years. In utero, IgM is able to be formed after 19–20 weeks gestation in response to exposure to certain antigens, such as the TORCH organisms. IgM protects against blood-borne infections and is the main immunoglobulin produced in the first four weeks of life. Half the adult value of IgM is reached by six months of age (Blackburn 2007).
Cell-mediated immunity
• Lymphocytes—at birth, T cell numbers are similar to those of adults but their function is decreased for approximately three to six months (Blackburn 2007). ‘The thymus gland, where lymphocytes are produced, is relatively large at birth and continues to grow until eight years of age’ (Farrell & Sittlington 2003b, p 730).
• Polymorphonuclear neutrophils and complement—immaturity of the polymorphonuclear neutrophils alters the inflammatory response and phagocytosis in neonates. Neonatal polymorphonuclear neutrophils are structurally more rigid, respond more slowly to antigen stimulus, move more slowly to the antigen site and are less efficient at aggregation and phagocytosis. Neutrophil function gradually matures through childhood.
Low levels of complement components contribute to decreased opsonisation capability. Serum complement levels increase to adult values by 6–18 months (Blackburn 2007). Nevertheless, Blackburn (2003) reports that neutrophils act normally in neonates when bacterial numbers are not too large and the baby is not stressed (e.g. by perinatal asphyxia). However, significantly reduced bacteriocidal activity for Gram-positive and Gram-negative organisms has been shown in stressed babies (Blackburn 2007).
Clinical point
Prevention and assessment of neonatal infection
• Practise standard precautions at all times.
• Teach parents to prevent infection through general hygiene measures such as hand washing, keeping equipment used for the baby in closed containers, and by encouraging common-sense precautions such as keeping the baby away from people with colds etc.
• Recognise any risk factors for development of infection and monitor for signs of onset.
• Understand the subtle signs of infection in neonates and particularly note that fever is rarely a sign of infection.
• Know and teach parents the signs of illness in their baby.
• Understand normal neonatal laboratory findings and recognise changes that are associated with neonatal infection.
Gut defence mechanism
In humans the gastrointestinal (GI) tract is part of the natural immune system. Defences in the GI tract include acidity, digestive enzymes that break down large molecules, and secretory IgA that lines the small intestine (Varney et al 2004). At birth, the epithelial lining of the GI tract is immature and relatively permeable. Development of the epithelium and the mucosal surface as an impenetrable barrier against pathogenic microorganisms and other antigens is known as gut closure. Gastric acid, peristalsis and the mechanical barrier properties of the gut mucosal surface are some non-immune factors that contribute to the gut defence mechanism (Blackburn 2007).
Role of breast milk in gut colonisation
Breastfeeding promotes gut colonisation because it provides a large amount of secretory IgA and stimulates the proliferation of intestinal enzymes. Colostrum and breast milk contribute to the development of protective gut flora by creating an acidic enteral environment, which favours growth of Lactobacillus and Bifidobacterium, thereby preventing growth of acid-sensitive organisms such as Bacteroides and enterobacteria. Breast milk also contains the protein lactoferrin, which binds with unabsorbed iron in the intestine, making it unavailable for bacterial metabolism (Blackburn 2007).
While there are fewer differences in gut colonisation when formula closely resembles breast milk, an alkaline environment is produced when babies are fed formula. In an alkaline environment, enterobacteria outcompete bifidobacteria, resulting in Gram-negative enterococci becoming the dominant organism in the intestine (Blackburn 2007).
Renal system
At birth the kidneys are structurally complete but functionally immature. There are limitations in the glomerular filtration rate capability and the ability to concentrate or dilute urine. Newborn babies have little renal reserve to cope with increased levels of solutes, which may occur in physiologically stressed or ill neonates (Michaelides 2004a; Novak 2005a; Varney et al 2004). The total body water content in newborn babies is a larger proportion of body fluid than in adults. The shift of intracellular fluid into the extracellular compartment after birth results in a diuresis that causes loss of 5%–10% of birthweight over the first week (Novak 2005a).
(Michaelides 2004a; Novak 2005a; Varney et al 2004).
When the bladder becomes full it is palpable abdominally. The kidneys may also be palpable. On micturition the direction and force of the stream of urine should be noted (Michaelides 2004a; Novak 2005a; Varney et al 2004).
Gastrointestinal system
Mouth
The neonate’s oral mucous membranes are pink and moist, and both the hard and soft palate are complete. The teeth are buried in the gums, but occasionally a tooth may be present in the mouth. Small epithelial retention cysts, called Epstein’s pearls, are sometimes found along the midline of the palate and occasionally elsewhere in the mouth. They disappear over time. The sucking pads in the cheeks give a rounded, full appearance to the face (Blackburn 2007; Michaelides 2004a; Varney et al 2004).
Swallowing and sucking
At birth the term baby has mature gag, cough, suck and swallow reflexes. As well as sucking fingers and thumbs in utero, the fetus swallows amniotic fluid from 10–14 weeks gestation to prepare the oesophagus to move food into the stomach by peristalsis. The swallowing reflex is mature at approximately 34 weeks gestation. The mature pattern of coordinated sucking–swallowing–breathing is seen as three to four bursts of sucking–swallowing followed by a pause for breathing. This pattern may not appear fully synchronised for a day or two after birth. The burst–pause pattern of sucking, swallowing and breathing seems to occur earlier in breastfed babies. Neonates cannot draw food from the lips to the pharynx, and therefore the nipple is drawn deeply into the mouth to enable suckling to occur (Blackburn 2007; Michaelides 2004a; Varney et al 2004).
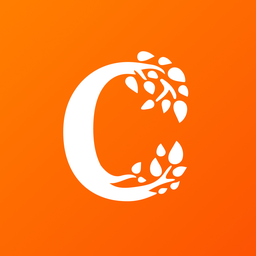
Full access? Get Clinical Tree
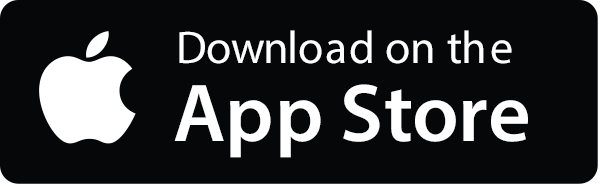
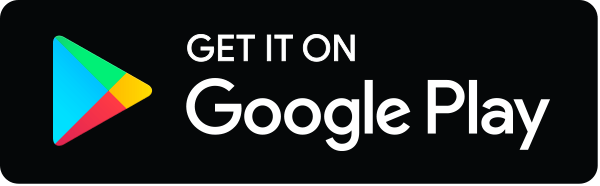