Chapter 6 Respiratory Health Breakdown
When you have completed this chapter you will be able to
INTRODUCTION
This chapter reviews the key aspects of respiratory system anatomy and physiology. Using a case study approach, the chapter examines respiratory health breakdown from three clinical categories: infective, traumatic and chronic problems. Important nursing considerations are discussed in relation to the case studies. Early recognition and intervention in the sequelae of respiratory problems are essential to improve patient outcomes and reduce morbidity and mortality. Table 6.1 highlights the breakdown of patient problems into clinical categories and common respiratory conditions. Continued deterioration in any of these conditions can lead to respiratory failure, which may develop rapidly even in the patient with little or no pre-existing lung pathology.
TABLE 6.1 RESPIRATORY HEALTH BREAKDOWN
Clinical Category | Common Conditions |
---|---|
Traumatic | |
Infective | |
Chronic |
Lung cancer is also a common disease of the respiratory system – it is the leading cause of male deaths and the second leading cause of female deaths from malignant disease in the world. Cigarette smoking is the predominant cause of lung cancer (80–90%), and is dose-response related. The common lung cancers include squamous cell; adenocarcinoma; small cell (oat cell) carcinoma; mesothelioma; and Kaposi’s sarcoma. Further discussion about cancers is provided in Chapter 14.
WHAT MAKES UP THE RESPIRATORY SYSTEM?
The primary function of the respiratory system is to provide oxygen (O2) for all metabolic processes to the body’s cells and tissues (oxygenation), and to remove carbon dioxide (CO2) from the gaseous waste products of metabolism (ventilation)1.
Anatomically, the respiratory system is divided into the upper and lower respiratory tracts. The key structures in the upper respiratory tract include the nasal cavities, the pharynx and the larynx, within the head and neck. The main function of the upper respiratory tract is to warm, filter and humidify inspired air. The nasal cavities are comprised of highly vascularised tissue consisting of pseudo-stratified, ciliated columnar epithelium. These structures provide a large surface area for heat and water exchange2. The pharynx is a funnel-shaped passageway and is shared by the respiratory and digestive systems. The larynx (voice box) connects the pharynx with the trachea, and contains cartilages with the epiglottic cartilage at the top. The epiglottis serves to create the cough reflex and protect the lower respiratory tract from aspiration of anything other than air. The largest cartilage is the thyroid cartilage (sometimes called the Adam’s apple). Three pairs of cartilage comprise the vocal cords. The last cartilage is the cricoid cartilage, which connects the thyroid cartilage and the trachea.
The lower respiratory tract includes the trachea and lungs (which include the bronchi, bronchioles and alveoli). A large portion of the lower respiratory tract is encased in and protected by the thoracic cage, and the muscles of ventilation, namely the diaphragm and the intercostal muscles (see Figure 6.1).
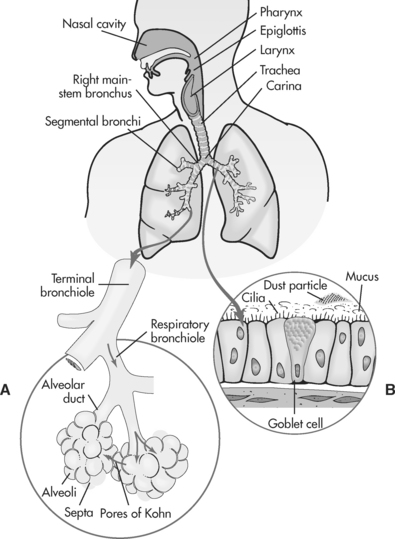
FIGURE 6.1 Structures of the respiratory tract
Source: Redrawn from Price, SA & Wilson, LM. 2003; Pathophysiology: Clinical, Concepts of Disease Processes (6th ed.), Mosby, St Louis, Fig. 35.1, p. 542.
The thorax or chest cavity contains the lungs, pleura and muscles of respiration. The lungs are cone-shaped organs, located on either side of the heart within the chest cavity. They contain part of the trachea, the main bronchi and bronchioles and alveoli. There are three distinct lobes of the right lung, and two lobes in the left lung. The pleura are serous membranes that cover the lungs and line the thoracic cavity. The function of the pleura is to act as a lubricating surface to enable the lungs to expand easily with respiration. The primary muscles of respiration are the diaphragm and the external intercostal muscles. The diaphragm is supplied by the phrenic nerve, which originates from the spinal cord at the level of the third cervical vertebrae. The diaphragm accounts for 75% of the change in thoracic volume during inspiration1. The external intercostal muscles are located between the ribs. Contract of these muscles during inspiration elevates the ribs, increasing thoracic volume.
PULMONARY BLOOD SUPPLY
The lungs have two different types of blood supply: pulmonary and bronchial. In pulmonary blood supply the lungs receive the body’s entire circulation volume each minute. The blood supply to the lungs begins at the pulmonary trunk, which stems from and receives deoxygenated venous blood from the right side of the heart. The pulmonary artery divides into the right and left main pulmonary arteries to supply the right and left lungs. These arteries continue to divide and subdivide until they form a pulmonary capillary network around each alveolus. It is here that diffusion of gases occurs. Following gas exchange, oxygenated blood is returned to the left side of the heart through the pulmonary veins2. Bronchial blood supply to the lungs commences with the bronchial arteries, which arise from the thoracic aorta. The bronchial arteries supply oxygenated blood to the bronchi and lung parenchyma. The majority of bronchial venous return is via the azygos vein to the left atrium.
NERVOUS CONTROL OF RESPIRATION
Respiration is an automatic function needing no conscious awareness. The respiratory centres in the medulla oblongata and upper pons in the brain stem control the stimulus to determine the rhythm of breathing. Neurones in the medulla oblongata control the cyclical pattern of inspiration and expiration. Excitatory impulses are transmitted to the diaphragm and external intercostal muscles to contract and commence inspiration. Two other areas in the respiratory centre of the brain stem assist in modifying the rhythm of breathing – the apneustic centre extends inspiration and the pneumotaxic centre limits or controls the time for inspiration. These centres work together to control the depth of respiration in response to the body’s needs3.
CHEMICAL CONTROL OF RESPIRATION
The respiratory system maintains normal amounts of oxygen, hydrogen ions and carbon dioxide within a dynamic state reflected by the following equation of the bicarbonate–carbonic acid buffer system2:
This equilibrium is controlled through the rate of breathing. Abnormal states such as hypoxia, acidaemia and hypercapnoea act to stimulate central and peripheral chemoreceptors. Central chemoreceptors are located in the respiratory centre in the brain stem and peripheral chemoreceptors are located in the bifurcation of the common carotid arteries and the arch of the aorta3. The primary chemical stimuli in breathing are hydrogen ions and CO2. Central chemoreceptors respond to hydrogen ion concentration and peripheral chemoreceptors respond to hydrogen ions and CO2. Chemoreceptors transmit impulses to the medulla oblongata to increase ventilation. Consequently, ventilation increases when hydrogen ion concentration rises and decreases when hydrogen ion concentration falls4.
MECHANICS OF BREATHING
Air moves in and out of the lungs during inspiration and expiration because of a pressure difference between the pressure inside the lungs (intrapulmonary pressure) and the pressure outside (atmospheric pressure)1. Atmospheric pressure at sea level is 760 mmHg standard temperature and pressure, dry (STPD). For inspiration to commence, the respiratory centre sends an excitatory stimulus to the diaphragm and the external intercostal muscles to contract. Contraction of the diaphragm lengthens the thoracic cavity. Contraction of the external intercostal muscles widens the thorax. This creates a pressure difference, as the thorax is now larger and intrapulmonary pressure has decreased to 758 mmHg. Air moves into the lungs from the higher external area of pressure to a lower area of internal pressure. At the end of inspiration, the muscles relax and the thorax becomes smaller. This increases the intrapulmonary pressure to 762 mmHg, which exceeds atmospheric pressure and air moves out of the lungs from a higher area of internal pressure to a lower area of external pressure.
GAS EXCHANGE AND TRANSPORT
Oxygen and carbon dioxide are exchanged at the alveolar capillary membrane by the process of diffusion. Oxygen moves from an area of high concentration (alveolar gas or atmospheric air) to an area of lower concentration (pulmonary capillary). Carbon dioxide moves from an area of high concentration (pulmonary capillary) to an area of lower concentration (alveoli). At cellular level O2 moves from the capillary to the tissues (cells) down a diffusion gradient. The pressure of O2 within the capillary is approximately 40 mmHg and within the cells is approximately 5 mmHg. The CO2 diffusion gradient is opposite to that of O2. At cellular level, CO2 moves from the tissues (cells) to the capillary. The pressure of CO2 within the cells is approximately 60 mmHg and within the capillary is approximately 1 mmHg.
Oxygen is transported in two ways: 97–99% is bound to haemoglobin (Hb), which is measured clinically by oxygen saturation (SaO2); 1–3% is dissolved in plasma5, which is measured clinically by arterial blood gas analysis (ABG) specifically the partial pressure of oxygen dissolved in arterial blood (PaO2). The oxygen-haemoglobin dissociation curve reflects the affinity of oxygen to the haemoglobin molecules. The curve demonstrates that there is not a linear relationship between PaO2 and haemoglobin-oxygen saturation (SaO2). This is particularly important at the critical level where PaO2 drops dramatically when compared to the SaO2 – while a 95% SaO2 equates to a PaO2 level of 80 mmHg, an SaO2 of 90% reflects a clinically significant drop in PaO2 to 60 mmHg (a state of hypoxaemia)2. The nursing considerations related to this issue are discussed later in the chapter. The PaO2 level is important for optimal oxygenation as it exerts a pressure to enable O2 to diffuse across capillary membranes to the cell6.
SUMMARY OF ANATOMY AND PHYSIOLOGY
Following are the key concepts:
ACUTE RESPIRATORY FAILURE
Urden, Stacy and Lough define acute respiratory failure (ARF) as
a clinical condition in which the pulmonary system fails to maintain adequate gas exchange4 (p. 551).
WHAT DOES ACUTE RESPIRATORY FAILURE MEAN?
The degree of failure is most commonly measured by continuous SaO2 bedside monitoring or through ABG analysis. If acceptable values of pH, PaCO2 and PaO2 are not maintained, the respiratory system is said to be in failure. Unlike other body system failures, it can be clinically difficult to offer definitive laboratory values on when respiratory failure does and does not commence – any deterioration is along a continuum, and the patient’s clinical presentation and underlying or pre-existing condition will be the best guides. All data, including subjective clinical history, clinical assessment and objective laboratory values, must be taken into account. As a guide for the clinician, the following values for respiratory failure are suggested:
Other laboratory criteria that may be helpful in determining acute respiratory failure are pH levels indicating acidaemia and an SaO2 of less than 90%7,8.
CAUSES OF ACUTE RESPIRATORY FAILURE
Failure of the respiratory system may be attributed to a variety of causes, some common, others uncommon. In order to breathe effectively and oxygenate tissues, a number of key systems need to be working effectively. To recall all the varied causes of respiratory failure, it is helpful to relate the causes to the anatomical reference points involved in respiration. Table 6.2 summarises some of the common causes of acute respiratory failure by linking clinical conditions to a location of the injury or malfunction.
TABLE 6.2 CAUSES OF ACUTE RESPIRATORY FAILURE
Location of Injury/Malfunction | Clinical Condition |
Respiratory centre in the brain stem | Direct trauma, infection, vascular lesions, brainstem lesions, drug overdose, central sleep apnoea |
Spinal cord | Cervical lesion or trauma |
Motor nerves | Guillain-Barré syndrome |
Neuromuscular junction | Myasthenia gravis, botulism |
Chest wall | Kyphoscoliosis, ankylosing spondylitis |
Pleura | Pneumothorax, haemothorax, tension pneumothorax, pleural effusion, rib fractures, flail segment |
Lung parenchyma (bronchioles, alveoli) | Pulmonary infection [viral, bacterial, fungal], aspiration, inhalation of toxins [smoke, chemicals], pulmonary oedema, cancer, acute respiratory distress syndrome (ARDS) |
Pulmonary circulation | Pulmonary embolism, primary pulmonary hypertension |
Source: dapted from Wilkins, Stoller & Scanlan3; Urden, Stacy & Lough4.
TYPES OF ACUTE RESPIRATORY FAILURE
WHAT IS THE PATHOPHYSIOLOGY OF ACUTE RESPIRATORY FAILURE?
There are four essential mechanisms identified in the pathophysiological sequelae of acute respiratory failure: alveolar hypoventilation; ventilation/perfusion (V/Q) mismatch; physiological shunt; and diffusion impairment9:
Not all gas inspired with each breath reaches the alveoli to participate in gas exchange. A portion is contained in the larger airways and upper respiratory tract and does not participate in gas exchange – this is called anatomical dead space. Anatomical dead space is estimated at 150 mL per 500 mL inspired breath. This dead space needs to be taken into account when estimating alveolar ventilation, or the amount of gas participating in gas exchange10. Alveolar minute ventilation can be represented as follows:
However, the relationship of alveolar ventilation to pulmonary perfusion varies considerably throughout the lung and is dependent upon a number of factors, such as pressure gradients affecting both ventilation and perfusion, and patient position (erect, supine or prone). In the upright adult person, the apices of the lung receive moderate ventilation but little perfusion (the V/Q here is > 0.8). Conversely, the bases of the lung in this scenario receive more perfusion and less ventilation (therefore, the V/Q is < 0.8). This is more balanced in the mid portion of the lung where, due to pressure equalising, ventilation and perfusion are evenly distributed2,10.
The pathophysiology of a mismatch in ventilation and perfusion results in systemic hypoxaemia. The types of V/Q imbalances that may occur include: 1) low-ventilation/ perfusion ratio – where perfusion exceeds ventilation, a physiological shunt is said to be present (see next section for further discussion); and 2) high ventilation/perfusion ratio – when ventilation exceeds perfusion, dead space develops since there is an overabundance of gas that is not able to participate in gas exchange – this can be caused by pulmonary embolism.
Theoretically, the tension of oxygen leaving the pulmonary capillary (PaO2) would be equal to the tension of oxygen in the alveoli (PAO2). However, physiologically the PAO2 (alveolar oxygen tension) is approximately 110 mmHg and the PaO2 (arterial oxygen tension) is 100 mmHg. This normal physiological difference of 10 mmHg is attributed to an anatomical shunt due to: 1) exiting of blood from lung regions with low ventilation in proportion to perfusion; 2) drainage of venous blood from the bronchial circulation into the pulmonary veins; and 3) drainage of some coronary venous blood directly into the left atrium by the thebesian veins4.
Clinically, a normal A-a gradient is less than 15 mmHg. If the A-a gradient is greater than 15 mmHg, this indicates underlying respiratory pathology, such as: 1) intracardiac shunts (atrial septal defects and ventricular septal defects); 2) pulmonary arteriovenous (AV) malformations; and 3) pulmonary disease when the alveoli are filled with fluid or exudate (pulmonary oedema, pneumonia, aspiration)11.
For diffusion to take place rapidly and effectively the diffusion membrane must have certain characteristics: 1) be very thin to facilitate rapid diffusion of molecules – all four layers of the alveolar capillary membrane are only 0.2–0.6 mcm thick2; 2) cover sufficient area to allow for enough exchange of gases – each lung contains 350 million alveoli, providing a surface area of 70 m2 for gas exchange; and 3) present a minimal diffusion pathway – the integrity of the diffusion membrane9 requires it to be free from accumulation of fluid or other substances that may impair gas exchange. The alveoli contain alveolar macrophages, which are phagocytic cells inside the alveoli that clear bacteria and other material invading the alveoli. Some causes of diffusion impairment are fluid accumulation from pulmonary oedema, and mucous and inflammation from pneumonia9.
WHAT DIAGNOSTIC INVESTIGATIONS ARE APPROPRIATE?
A thorough health history and physical assessment of the respiratory system often uncovers problems worthy of further investigation or confirmation8. The recommended reading by Finesilver (2001) offers a thorough overview of respiratory health history and physical assessment. Adjunctive assessment tools, such as pulmonary function tests, pulse oximetry, arterial blood gas results and chest X-rays, can often provide valuable quantitative information regarding the respiratory system.
PULMONARY FUNCTION TESTS
Pulmonary function tests are designed to quantify respiratory function according to gender, age and body size, and form an essential part of respiratory assessment. Pulmonary function tests serve many purposes, such as preoperative evaluation, evaluating lung mechanics, diagnosing and monitoring pulmonary diseases, and monitoring response to therapy12. There are four areas to a complete set of pulmonary function tests: lung volumes (this covers spirometry and vital capacity); mechanics of breathing (measures flow of gas and lung compliance and resistance); diffusion of gases (refers to ventilation/perfusion scanning); and arterial blood gases. Commonly, bedside spirometry includes assessment of forced expiratory volume in 1 second (FEV1) and forced vital capacity (FVC), to evaluate respiratory status or in response to treatment. Other lung mechanics are usually measured in specific pulmonary function laboratories.
ARTERIAL BLOOD GASES
The goal of interpreting arterial blood gas (ABS) analysis is to assess how effectively the body is maintaining homeostasis, keeping the pH of the body within normal limits. Table 6.3 reviews the normal values for each of the ABG components.
Changes differing from these normal values will reflect changes in the acid-base balance6. For example, a pH less than 7.4 indicates acidosis; whereas a pH greater than 7.4 indicates alkalosis. The PaCO2 represents the partial pressure of carbon dioxide dissolved in arterial blood. Arterial carbon dioxide levels are indicative of ventilation. Thus PaCO2 levels are used to measure respiratory acid-base balance. Bicarbonate (HCO3−) is the main chemical buffer in plasma. Bicarbonate levels are used to measure metabolic acid-base balance. Base excess refers to the quantity of strong acid or base required to restore the pH to 7.4. A positive value of BE (e.g., >+2) indicates an excess of base (or deficit of acid), while a negative value (e.g., <−2) indicates a deficit of base (or excess of acid).
The steps in interpretation of ABGs are13:
OXYGEN SATURATION (SAO2)
The use of pulse oximetry has become a standard tool in most clinical settings14. A pulse oximeter measures the percentage of haemoglobin molecules saturated with oxygen (SaO2). The normal value for SaO2 is 95–99% on room air15. As noted earlier, a limitation of pulse oximetry is that the SaO2 does not always accurately reflect oxygenation (PaO2), particularly when SaO2 falls below 90%. A number of clinical and technical factors also affects the accuracy of pulse oximetry: 1) poor peripheral pulsatile states (mean BP ≤ 50 mmHg, narrow pulse pressure, vasoconstriction); 2) other physiological states (e.g., anaemia, carboxyhaemoglobin); and 3) technical factors (e.g., motion artifact, external light interference, intravenous dyes)15.
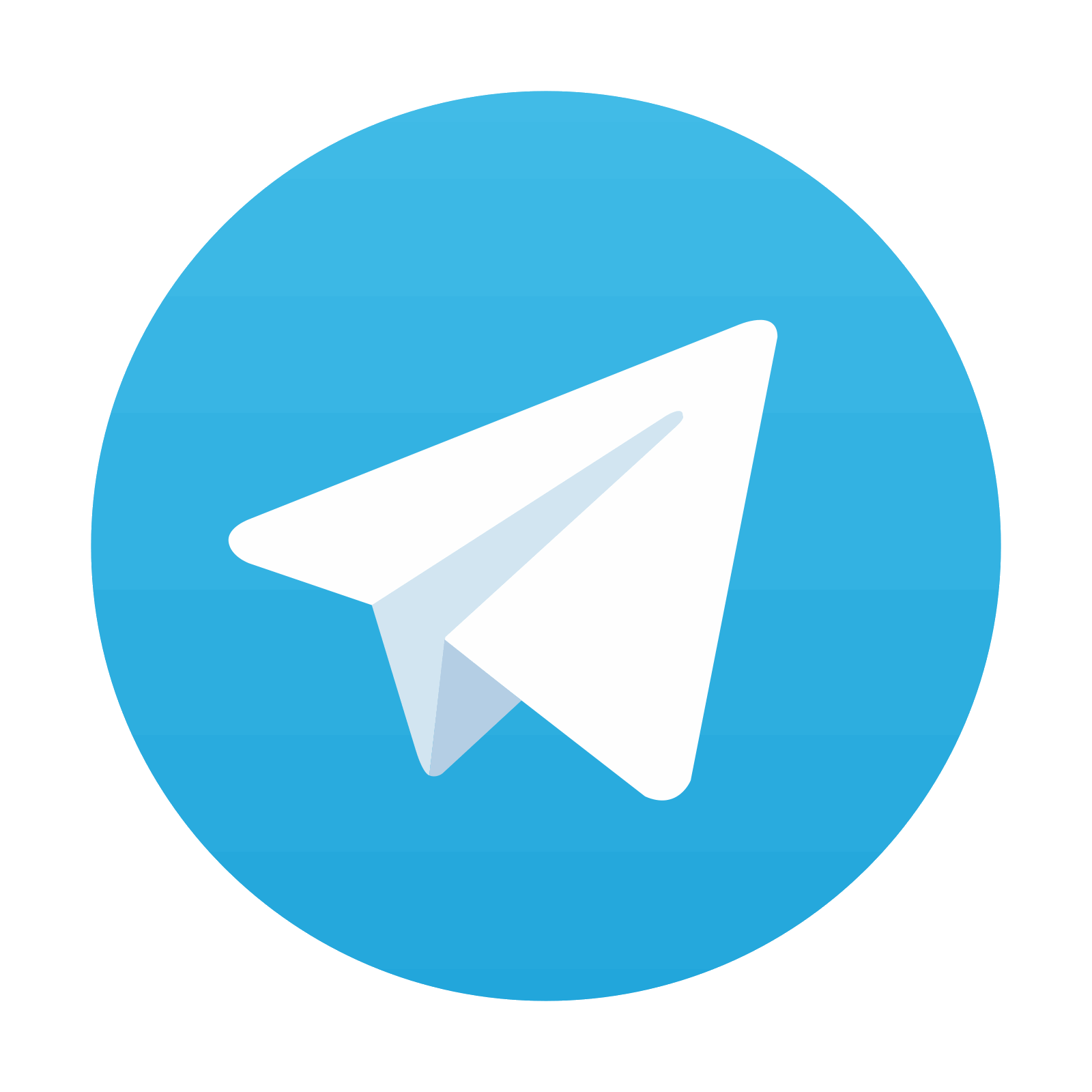
Stay updated, free articles. Join our Telegram channel
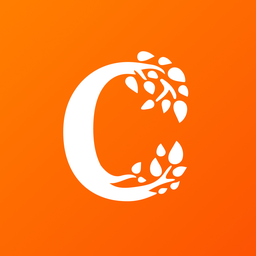
Full access? Get Clinical Tree
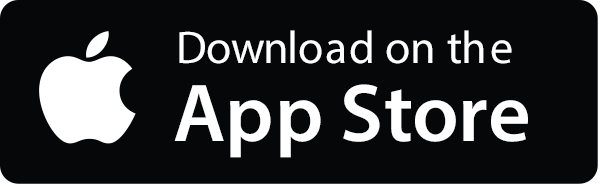
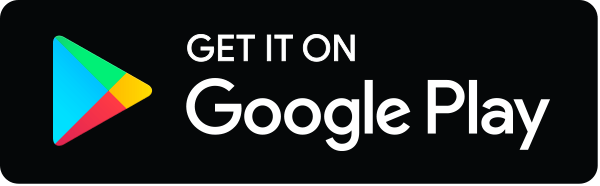