Stages of labour
Fig. 13.2
Fig. 13.3
The uterus at term
Uterine growth in pregnancy
Uterine muscle organization
Fig. 13.4
The myometrium
The role of calcium
The control of myometrial contractions
Gap junctions
Fig. 13.7
Uterine contractions and quiescence
The cervix
Fig. 13.8
Assessment of cervical effacement
Bishop’s Score
0
1
2
Station of presenting part
−3
−2
−1
Position of cervix
Posterior
Mid
Anterior
Consistency
Firm
Soft
Very soft
Length
3–4 cm
1–2 cm
<1 cm
Dilation of cervix
0
1–2 cm
>2 cm
Total=
(Bishop’s score)
Initiation of parturition
Animal models of parturition
Initiation of parturition in humans
Hormonal changes associated with parturition in humans
The role of the fetal pituitary–adrenal axis
Cortisol
Progesterone:oestrogen ratio
Corticotrophin-releasing hormone
Other factors
Drug/Treatment
Effect
Notes
Treatment of preterm labour (tocolytic agents)
Progesterone and related compounds
May relax uterus or block inflammatory pathways
Possibly reduce late preterm birth but not associated with reduced perinatal mortality or morbidity
β-Adrenergic agonists (betamimetics or ‘β-agonists’), for example ritodrine, terbutaline, fenoterol
Inhibit uterine contractions
Effects often short-lived; unpleasant side-effects (cardiovascular, metabolic and neuromuscular)
Magnesium sulphate
Inhibits myometrial contractility by competing with calcium entry and inhibiting actomyosin interaction
Limited usefulness as a tocolytic agent but useful as cerebroprotective agent
Oxytocin receptor antagonists, for example atosiban,barusiban
Competitive inhibition of oxytocin
Clinical trials taking place; potentially useful as oxytocin receptors have limited distribution. Some side effects as acts on vasopressin receptors
Prostaglandin synthase inhibitors, for example indomethacin
Inhibit preterm contractions
Serious neonatal complications in infants born <30 weeks
Reduce connectivity between myocytes
Adverse effects on fetal renal function, associated with oligohydramnios
Calcium-channel blockers, for example nifedipine
Decrease intracellular calcium, cause uterine relaxation
May affect placental and uterine blood flow; cardiovascular side-effects (hypotension and tachycardia); nonsteroidal anti-inflammatory agents
Alcohol
Risk of aspiration, intoxication, depression and incontinence
Treatment of post-term labour (methods of induction)
Oxytocin
Used to augment labour; oxytocin has little effect on unripe cervix
Production of endogenous oxytocin can be increased by nipple stimulation
PGF2 agonists, for example dinoprostone, Cervidil, Prepidil
Cervical ripening, induction of labour
Effects depend on expression of receptors
Misoprostol (Cytotec)
Synthetic PGE1 analogue; used to reduce acid in the stomach
Can cause the uterus to contract; nausea and diarrhoea
Mifepristone (RU-486; Mifegyne)
Antiprogestin
Primarily used to induce first trimester abortion
Signal amplification
Prostaglandins
Oxytocin
Stay updated, free articles. Join our Telegram channel
Full access? Get Clinical Tree
Physiology of parturition
While working in Africa, Zara had been able to assist several of her African friends during childbirth, all of which had occurred at home and attended by mostly female relatives who had informed Zara that they would only call the local midwife if they felt things were going wrong. All her friends had had uncomplicated deliveries and Zara felt quite privileged to have witnessed childbirth in such a different way to how it is presented within most modern Western societies.
Zara is keen to have a waterbirth at home and wants to avoid all forms of pain relief. She also wants her sister and some of her close friends who are also heavily pregnant to be present if possible. As Zara has had all her antenatal care from her midwife, she particularly wants her midwife to care for her in labour, especially as her midwife has extensive experience of waterbirth.
• What factors do you think will be a positive influence on enabling Zara to have a normal birth?
Preterm birth is the predominant cause of perinatal morbidity and mortality in developed countries. Most early neonatal deaths, that are not associated with a lethal deformity, are associated with prematurity and preterm infants have an increased risk of complications not just in the neonatal period but in the long-term (Saigal and Doyle, 2008). Long-term consequences include cognitive and motor neurodevelopment disabilities that include cerebral palsy, mental retardation, deafness, blindness, learning disabilities, chronic lung disease and possibly an increased risk of disease in adult life. The shorter the gestation, the poorer the prognosis is. Even though very low-birth-weight babies (i.e. those born below 1000 g) may now survive, it is generally associated with rates of morbidity and much emotional stress for their parents, as well as a high financial burden on neonatal intensive care units. There are also long-term health and education costs associated with physical and mental handicap and neurodevelopmental complications. It can be argued that one of the principal objectives of obstetrics is to reduce preterm labour. The survival rate of premature infants born alive with borderline viability has improved over the last decade (Field et al., 2008), however improved survival rates have not been matched by a proportional decrease in the incidence of disability (Stephens and Vohr, 2009).
There are three categories of causes of preterm birth: iatrogenic or indicated, where complications of pregnancy such as eclampsia, pre-eclampsia or intrauterine growth retardation, enforce obstetric intervention and the deliberate induction of premature delivery (30–35% of cases); preterm premature rupture of (fetal) membranes (PPROM), which may be associated with infection (25–30% of cases); and spontaneous or idiopathic preterm labour (40–45% of cases; Goldenberg et al., 2008). The failure of spontaneous labour is also not well understood; prolonged pregnancy (gestation >42 weeks or 294 days) is also associated with increased fetal morbidity and mortality.
The factors controlling the transition from one state to the other are not well understood but are very important both in determining the possible causes of preterm labour and in understanding how to induce labour successfully without eliciting fetal distress. The control of the onset of human (and primate) parturition remains elusive. There are marked differences between human and other mammalian species in the cascade of factors leading to parturition. Humans have a very high rate of premature birth compared with other species. For most domestic and laboratory animals the duration of gestation is remarkably constant; for example, there is less than 1% variation in sheep (Jenkin and Young, 2004). Theoretically, the length of gestation does not matter to the mother. The crucial aspect is that the baby can survive from birth. It seems plausible, therefore, that the fetus controls the length of gestation. Certainly, in some experimental animals, for example sheep, there is proven fetal involvement in the timing of labour but it is difficult to obtain such evidence in humans.
In other species, it seems that the same signal controls fetal maturation and triggers the onset of labour so parturition is synchronized. In humans, the two pathways seem to be separable (Fig. 13.1). The human fetus appears to undergo lung maturation 4–6 weeks before labour, unlike other species in which the signals initiating labour also stimulate fetal organ maturity. It is not clear why the events leading to parturition should be so complex in the human; however, it seems plausible that a variable length of gestation is advantageous. The complexity of control of parturition in humans might allow a transfer of control from mother to fetus. Thus, in early pregnancy, it may be physiologically expedient for the mother to terminate the pregnancy if it is harmful for her long-term health to continue. Spontaneous termination of a pregnancy that is unlikely to be completed, for example because maternal nutrient intake is insufficient, prevents needless maternal investment. Later in gestation, once the fetus is mature enough to survive, fetal control of parturition would allow the fetus to remain in the uterus if the environment was favourable. The fetus could respond to stress by switching from cell division and growth to accelerated maturation and earlier initiation of parturition. This would suggest that intrauterine growth restriction is part of an adaptive response to fetal stress which increases survival as long as the stressor is not too early or too severe. It is not surprising, therefore, that many of the signals involved in parturition are also involved in physiological stress responses. Midwifery and obstetric management of women in labour is often interventionist. This chapter covers the physiology of parturition; for information about clinical management, readers are referred to midwifery texts in the list of further reading.
From a clinical point of view, labour is often divided into three stages (Fig. 13.2). However, physiologically there is no abrupt transition between stages. The events leading to the onset of labour are gradually and inconspicuously initiated earlier in the pregnancy, and the three stages overlap. The first stage is that of progressive cervical dilatation, timed from the onset of regular coordinated contractions accompanied by progressive effacement (thinning) and dilatation of the cervix. The end of this stage is marked by the full dilatation of the cervix as the uterine contractions pull the entire tissue of the cervix upwards until it becomes incorporated into the lower uterine segment, continuous with the uterine walls. This stage lasts an average of 12–14 h in primigravidae, but tends to be shorter in multigravidae. The second stage is fetal expulsion, from full cervical dilatation until the delivery of the baby. The contractions are strong and aided by the respiratory muscles. The second stage may take over an hour in primigravidae and as little as a few minutes in multigravidae. The third stage of labour involves separation and complete expulsion of the placenta and membranes, and control of bleeding from the uteroplacental circulation. The return to the prepregnant state is described as the puerperium (see Chapter 14).
From a physiological point of view, it is useful to think of labour being related to phases of uterine myometrial activity. For most of pregnancy, the uterus is in phase 0, the quiescent phase. Under the influence of progesterone (the literal meaning of progesterone is ‘pro-gestation’, i.e. promoting and sustaining pregnancy), the uterus is relatively quiet and non-responsive to stimuli. Other factors involved in quiescence are prostacyclin, nitric oxide, relaxin, parathyroid hormone-related peptide, calcitonin gene-related peptide and vasoactive intestinal peptide (Terzidou, 2007). All these factors act to increase cAMP (or cGMP) and thus inhibit the release of intracellular calcium that is required for myometrial activity. In late pregnancy, the uterus changes from being quiescent (having a low level of muscle activity) to being activated; this is known as phase 1, the activation phase. This transition to activation is the initiation of labour; labour results from activation and then stimulation of the myometrium. The receptors and signalling pathways are modulated so they respond to contractile stimuli. Activation is partially stimulated by mechanical stretch of the uterus together with changes in signalling via endocrine and paracrine pathways, possibly resulting from an increased activity of the fetal hypothalamic–pituitary–adrenal (HPA) axis. Increased levels of oestrogen and CRH lead to an up-regulation of genes involved in contraction including genes for connexin 43, prostaglandins and oxytocin receptors. The increased production of oestrogen may be due to increased availability of fetally derived precursors. In the third phase of parturition, phase 2 – stimulation, the activated uterus is spontaneously excitable and responsive to uterotonins such as prostaglandins, oxytocin and CRH; it develops coordinated, effective and forceful contractions. The activation of the uterus in this stimulation phase is the start of a positive feedback loop whereby the initial signals become further amplified and the uterus becomes fully stimulated allowing progression of the first and second stages of labour. This phase is accompanied by inflammatory-like biochemical changes. There is increased synthesis of prostaglandins and cytokines and an influx of neutrophils which produce proteases which are involved in remodelling and ripening of the cervical tissue. The uterus is able to perform a remarkable mechanical effort to expel its contents – the baby, placenta and associated membranes and fluids – through the birth canal (Fig. 13.3). The final phase is the uterine involution phase.
The uterine muscle undergoes exceptional growth throughout pregnancy to accommodate the growing fetus. The prepregnant weight of the uterus is about 50 g in a nulliparous woman and about 60–70 g in a multiparous woman with a capacity of about 10 mL. During pregnancy, uterine size increases 20-fold, to about 800–1200 g and a capacity of about 5 L. Initially, the uterus grows by hyperplasia (increasing cell number). This is under the influence of oestrogen and, unlike later growth, occurs regardless of the site of implantation. By the 4th month, the uterine wall has thickened from 10 to 25 mm. Subsequent growth is due to hypertrophy and stretch stimulated by uterine distension. The uterine wall thins and the smooth muscle cells increase markedly in length (from 50 to 500 μm long and from 5 to 15 μm wide) as they accumulate contractile proteins. The increased overall size is accompanied by a change in uterine shape from a sphere to a cylinder. By term, the organization of the myometrial cells allows coordinated, strong and effective contractions to develop. The uterine muscle is innervated by adrenergic, cholinergic and peptidergic fibres, which are more abundant in the cervix and uterine tubes. The uterus also has many sensory nerves. By the third trimester, the uterine wall is thin, about 5–10 mm by term; the fetal movements are visible and the fetus can be palpated through the uterine wall (Blackburn, 2007). The uterus almost reaches the liver and displaces the stomach and intestine. The blood vessels in the non-pregnant uterus are extremely tortuous and coiled (see Chapter 2); this allows them to adapt to the increased requirements of the expanding uterine tissue. Uterine blood flow increases in pregnancy as the blood vessel diameter increases and resistance to flow decreases.
The uterus is predominantly comprised bundles each of 10–50 myometrial (smooth muscle) cells separated by connective tissue, formed of collagen and elastin. The distribution of the smooth muscle varies throughout the length of the uterus. The smooth muscle density is highest in the fundus of the uterus (an approximate ratio of smooth muscle fibres:connective tissue of 90:10) and gradually declines until the cervix where the ratio is 20:80. Associated with the myometrium are leukocytes which produce cytokines. Pro-contractile proteins such as oxytocin receptors and connexin 43 are preferentially expressed in the fundal region of the uterus. The isthmus, which forms the lower segment of the uterus, has lower smooth muscle content. The lower segment forms at about weeks 28–30 of pregnancy. Caesarean sections late in gestation are usually via the lower segment of the uterus (lower-segment caesarean section, LSCS), whereas the incision in an emergency ‘classic’ caesarean section earlier in pregnancy usually is on the midline of the uterus and is likely to dictate the method of delivery in future deliveries. Contractile strength is related to the proportion of smooth muscle (Petersen et al., 1991). Therefore, the upper part of the uterus contracts strongly and the lower segment, which has a diminishing proportion of muscle, contracts weakly and passively (see Fig. 13.10, p. 338).
The uterine muscle forms three distinct anatomical layers, which are more evident with the hypertrophy of the uterus that occurs in pregnancy (Fig. 13.4). The innermost layer has muscle mostly in a longitudinal orientation. The myometrium has more muscle fibres in the inner layer than it does in the outer layers (Terzidou, 2007). The outermost layer has longitudinal and circular fibres. The middle layer of uterine muscle has spiralling fibres and is particularly well vascularized. It is this middle layer that ensures the blood vessels in the uterus are occluded in the third stage of labour as the spiralling fibres contract around the blood vessels. The lower uterine segment has a high expression of CRH receptor type I which is involved in relaxation and of the enzymes involved in cervical ripening.
(Reproduced with permission from Sweet and Tiran, 1996.)
The myometrium is formed of myometrial cells embedded in a collagen-rich connective tissue matrix which has blood vessels interspersed within it. The cytoplasm of the myometrial cells or myocytes is packed with long random bundles of actin and myosin. Compared with skeletal muscles, the concentration of actin is higher and the myosin has longer filaments, which increases the maximum shortening of the contractile cells. Myosin is both a structural protein and an Mg-ATPase, an enzyme that can hydrolyse ATP and utilize the energy for movement. When ATP is hydrolysed (broken down), actin and myosin cross-bridges form so actin and myosin slide past each other, shortening the cell so that the muscle contracts (Fig. 13.5). Myosin is made up of two heavy chains forming the ATPase and two light chains, which bind calcium and undergo phosphorylation. Phosphorylation is the incorporation of a phosphate group catalysed by a kinase, in this case myosin light-chain kinase (MLCK) which effectively activates the protein. Factors that negatively affect myometrial contractility may be important in maintaining uterine quiescence through pregnancy; those that increase contractility may be important in facilitating the progression of labour.
Muscle contraction is triggered by a rise in the intracellular concentration of calcium ions. Electrical activity, calcium ion influx and development of myometrial tension are precisely synchronized (López Bernal, 2003). Calcium ions bind to the calcium-binding protein calmodulin (CAM) which regulates the activity of many of the intracellular enzymes generating a cascade of reactions leading to binding of actin and myosin (Wray et al., 2003). Binding of calcium to CAM forms a Ca–CAM complex which activates MLCK. MLCK is inhibited when calcium levels are low. The activated MLCK undergoes a conformational change which exposes the catalytic site so the MLCK can be phosphorylated and will interact with actin, initiating contractions. Removal of calcium results in dephosphorylation of myosin by myosin light-chain phosphatase and causes muscle relaxation. Smooth muscle contraction can therefore be increased either by activating MLCK or by inhibiting myosin phosphatase.
Calcium enters the myometrial cell from the extracellular fluid or is released from intracellular binding sites and organelles including the sarcoplasmic reticulum of the myometrial cells. Calcium released from the sarcoplasmic reticulum can prime contractions that need to be sustained by calcium influx. Voltage-operated calcium channels regulate contractility by allowing calcium entry. Calcium-activated potassium channels set the threshold of activation of the cell membrane. There is a good correlation between intracellular calcium concentration and the muscular force developed with ionized calcium (Ca2+) concentration increasing from approximately 100–500 nm during contraction. Uterotonins (substances that stimulate myometrial contractility), such as prostaglandins and oxytocin, increase calcium influx and mobilize intracellular calcium stores, therefore increasing intracellular calcium concentrations and MLCK phosphorylation and increasing myometrial activity. Agents that inhibit myometrial activity, such as progesterone, β-mimetics, relaxin and prostacyclin, decrease intracellular Ca2+ by promoting calcium uptake into the intracellular stores such as the sarcoplasmic reticulum so free calcium levels decrease and the uterine muscle relaxes. Calcium channel blockers, such as nifedipine, prevent calcium entry into the cells promoting relaxation of the uterus.
Intracellular calcium levels (and myometrial activity) are controlled by various receptors on the myometrial cell surface. Most hormones that affect myometrial contractility bind to receptor sites that are coupled to one of two G-proteins: Gαs or Gαq (see Chapter 3; Bernal et al., 1995). The G-proteins act as transducers between the receptor and the effector regulating the cellular response by coupling the receptor to different signal-generating enzymes within the cell. These enzymes, in turn, generate an amplifying cascade of second messengers. The G-proteins allow the myometrial tissue to respond to a large number of agonists with a limited number of effects, either relaxation or contraction (Fig. 13.6). One of the G-protein pathways (Gαq) is linked to the inositol phosphate pathway. Binding of agonists (uterotonins) to receptors coupled to this G-protein activates phospholipase C (PLC), generating inositol triphosphate and diacylglycerol. Inositol trisphosphate stimulates the release of calcium ions from the sarcoplasmic reticulum and diacylglycerol activates the enzymes protein kinase C and phospholipase A. The latter releases arachidonic acid from membrane phospholipids; arachidonic acid is the precursor of prostaglandins. The result is a rise in intracellular calcium and therefore smooth muscle contraction. The other G-protein pathway (Gαs) activates adenylate cyclase, an enzyme that generates cyclic adenosine monophosphate (cAMP) and inhibits release of calcium. Agonists that stimulate this pathway, such as β-adrenergic receptor agonists and prostacyclin, will cause decreased release of Ca2+ from intracellular Ca2+ stores and dephosphorylation of myosin (so MLCK cannot bind Ca–CAM) and thus uterine relaxation (Yuan and López Bernal, 2007).
The onset of regular uterine contractions is gradual. As pregnancy progresses, the resting potential of the myometrial cells falls so that action potentials are generated with greater ease. Initially spontaneous uterine contractility is inhibited but becomes significant by mid-gestation. At about 20–24 weeks, hardening (or ‘tightening’) of the uterus can be felt as muscle contractions start. Initially, uterine activity is mostly of low amplitude and high frequency, with peak activity at night. This circadian rhythm stops at about 3 weeks before delivery, and may be mediated by cortisol (Germain et al., 1993). At first, small groups of myometrial cells contract together causing small fluctuations in intrauterine pressure. As pregnancy progresses, adjacent cells begin to contract synchronously so the contractions become more coordinated. This increased coordination results from cell–cell coupling and is due to the formation of intercellular gap junctions.
Gap junctions are formed from bundles of proteins, called connexins, that align forming pore-like symmetrical channels protruding through adjacent cells, so allowing contact and communication. Gap junctions exist in other tissues that act together in a coordinated fashion, such as cardiac muscle and pancreatic islets. In the open state, gap junctions allow rapid transmission of signals such as electrical stimuli and second messengers such as calcium and inositol trisphosphate (Fig. 13.7). This means that depolarization and smooth muscle contraction in one cell are quickly communicated to adjacent cells so there is a spread of excitation and a synchrony of contractions.
There is an increase in the synthesis of gap junctional protein connexin 43 and the number of gap junctions increases during gestation to about 1000 per myometrial cell. Physiological regulation of connexins and formation of gap junctions is by prostaglandins and steroid hormones; oestrogen and some prostaglandins increase and progesterone and nitric oxide suppress gap junction formation (Garfield et al., 1988). Gap junction density can be determined by measuring electrical resistance of tissue. The resistance of human myometrium at term is about half of that in the non-pregnant uterus and much less than that in other smooth muscle (for instance bladder and stomach), which indicates that the cells of the pregnant myometrium are very well coupled. Gap junction formation increases prior to spontaneous labour, resulting in synchronization and coordination of high-amplitude high-frequency myometrial contractions so increased intrauterine pressure is generated (Neulen and Breckwoldt, 1994). This is why palpation especially near to term, can stimulates uterine tightening; palpation initiates the first stimulation of the uterus which then spreads throughout the myometrium. Often fetal movements will trigger uterine tightening for the same reasons. Suppression of gap junctions may be important at the time of implantation and in early pregnancy (Grummer and Winterhager, 1998).
The uterus exhibits spontaneous contractility. A biopsy specimen of uterine tissue, placed in a physiological solution, will contract involuntarily every 2–5 min without stimulation. During the menstrual cycle, three patterns of uterine contractility have been described (De Ziegler et al., 2001). At the beginning of the cycle (menstruation), all layers of the myometrium contract exerting anterograde (from fundus to cervix) expulsive forces; these contractions may be associated with painful cramps (dysmenorrhoea). During the receptive window, in the late follicular phase, uterine contractility involves only the inner layers of the myometrium and is gentle and not perceived by women; this facilitates retrograde (cervix to fundus) transport of sperm towards the uterine tubes where fertilization usually takes place (see Chapter 7). As progesterone levels rise after ovulation, the uterus reaches a stage of quiescence. In pregnancy, progesterone continues to increase and to suppress the rhythmic activity of the uterus. Progesterone decreases the expression of genes for contraction-related proteins, stimulates the relaxant pathways and suppresses the stimulatory pathways of the myometrium and inhibits the binding of oxytocin to its receptors (Mitchell and Taggart, 2009). Other inhibitors of uterine contractions early in pregnancy include relaxin, prostacyclin and nitric oxide, all of which increase intracellular cAMP and/or decrease intracellular calcium levels. It is also suggested that human chorionic gonadotrophin (hCG) inhibits myometrial contractility (Slattery et al., 2001).
However, the uterus is never completely quiescent. From about 7 weeks, the contractions, or ‘contractures’, are irregular, not synchronized and focal in origin; they have a very high frequency and a very low intensity (Wray, 1993). From mid-gestation, the contractions increase gradually in intensity and frequency until about 6 weeks before term when their intensity increases more markedly. The early Braxton Hicks contractions can be perceived but, although strong, they are not normally painful as the cervix remains closed. In labour, the contractions become synchronized, regular and more intense with increased duration. In primate models, in the few days before delivery, uterine contractions become synchronized during the night and disappear during the day until delivery. This pattern has not been observed in humans but women do have periods of active contractions which then cease suggesting there is also a degree of reversibility in the early stages of human labour (Smith, 2007).
The characteristics of the myometrium change markedly during pregnancy (Shynlova et al., 2009). In early pregnancy, there is a proliferative phase when the expression of IGF proteins is increased and an anti-apoptotic pathway is up-regulated. This is followed by a synthetic phase of growth and remodelling involving cellular hypertrophy and increased synthesis of extracellular matrix proteins. In the third phase the myometrial cells develop a contractile phenotype exhibiting increased excitability and sensitivity to calcium, spontaneous activity and enhanced responses to agonists. In the final phase of pregnancy, the myometrial cells are highly active and committed to labour. The cells are more excitable, there is increased connectivity between the cells and there are changes in the contractile proteins. In this phase, the myometrial cells actively participate in the inflammatory process by producing pro-inflammatory cytokines. There is a further stage of postpartum uterine involution (see Chapter 14).
The consistency of the cervix changes in pregnancy to become softer and compliant in preparation for labour. For most of pregnancy, the cervix is a rigid cylindrical structure about 4–7 cm long which forms a closed canal (Mitchell and Taggart, 2009) though in women who have had a previous vaginal delivery, the cervix may be slightly shorted with an internal diameter of about a centimetre. The cervix consists mostly of collagen fibrils, elastic connective tissue and blood vessels with some smooth muscle fibres. Connective tissue changes affect the whole uterus but are more evident in the cervix. The uterine contractions imposed on the softened cervix result in it changing shape. During the pregnancy, the role of the cervix is to act as a closure for the uterus containing its contents and protecting them from ascending infection. Prior to the delivery of the baby, the cervix loses its structural rigidity and is pulled by the uterine contractions so it changes from being a tubular closure to becoming a wide-funnelled canal with very thin edges that is continuous with the rest of the uterine structure. In primigravida women, this shape change occurs in two distinct stages.
The first stage of cervical change is effacement, where the cylindrical shape is transformed into a funnel, but the internal sphincter, or os, is still patent and closed. The longitudinal muscle fibres of the cervix shorten. The differential localization of the fibres means that the outer margins of the cervix develop more tension so maximum uptake of the cervix occurs at the lower end and the external os and softer cervical tissue move upwards into the lower uterine segment (Gee and Olah, 1993). During vaginal examination, a midwife might feel an ‘effacement ridge’ of the cervical tissue undergoing effacement (Fig. 13.8) the ridge is the dissipating form of the external os.
(Reproduced with permission from Sweet and Tiran, 1996.)
The second stage begins when full dilatation is reached (the edges of the internal os can no longer be felt); the uterus and vagina form one continuous ‘sleeve’ opening for the exit of the fetus. In multiparous women, the transition from one stage to the other is far less abrupt so effacement and dilatation occur simultaneously. Dilatation is due to the retraction or shortening of the upper part of the uterus, rather than pressure from the descending presenting fetal part. Therefore, if there is no effective presenting part, as in a transverse lie, cervical dilatation still occurs. The dramatic changes in the cervix result from a combination of structural changes in the tissue and forces exerted by the uterine contractions.
The cervix is predominantly composed of fibrous connective tissue plus some smooth muscle and fibroblasts together with blood vessels, epithelium and mucus-secreting glands. The rigidity of the cervix is related to its high content of collagen, particularly type I and type III collagen. There are two elements to cervical softening: increased vascularity and water content, and structural changes in the connective tissue. At term, 90% of the weight of the cervix is water. Connective tissue is formed of collagen fibres and elastin held together by an extracellular matrix, or ground substance. The ground substance is predominantly composed of proteoglycans, which coat the collagen fibres and modify their physical properties, determining the water content of the tissue. Hormones that promote cervical softening affect the composition of the ground substance. Prior to the onset of labour, there is increased expression and activity of matrix metalloproteinases (MMP) which leads to a progressive breakdown of the collagen matrix; the composition of the proteoglycans changes so that dermatan sulphate decreases and hyaluronic acid and glycosaminoglycans increase. Dermatan sulphate binds collagen fibrils tightly, whereas hyaluronic acid has a lesser affinity for collagen and attracts water. Although the proteoglycans are a minor constituent of the cervix, they have an amazing ability to bind water: 1 g of hyaluronic acid can bind about 1 L of water (Uldbjerg and Malstrom, 1991). The increased level of hyaluronic acid may act as a signal to activate resident macrophages and neutrophils to secrete interleukins. Interleukins increase prostaglandin activity and neutrophil migration and degranulation (releasing collagenase and elastase). Two other glycoproteins are also involved in cervical changes. Decorin binds and immobilizes the collagen fibres thus stabilizing the structure of the extracellular matrix in early pregnancy. Concentrations of decorin fall in late gestation. Fibronectin binds dermatan sulphate and collagen, protecting collagen from collagenase and stabilizing the extracellular matrix. Hyaluronic acid weakens the interaction of collagen with fibronectin.
The mechanical strength of the ground substance changes as the water content increases and the number of cross-links between elements of the connective tissue diminishes. The collagen increases in solubility and becomes disorganized and weakened (like a fraying rope) so it is more vulnerable to enzymatic digestion. Collagen is resistant to most proteases except collagenase from fibroblasts and neutrophil elastase. Amounts of neutrophil elastase in the cervix significantly increase at term. The association between intrauterine infection and premature labour may be linked to neutrophil infiltration and activation. The effects of oestrogen on cervical ripening are suggested to be mediated by insulin-like growth factor I (IGF-I; Stjernholm et al., 1996). Collagenolysis is a complex balance between availability of free collagenase and the inhibitory proteins. Connective tissue in the body of the uterus also changes at term altering uterine compliance. The level of elastin increases throughout the pregnancy. It provides the elastic recoil that coordinates the contraction–retraction cycle and is important in the return of the uterus to its normal shape after delivery.
Although the cervix has relatively little smooth muscle tissue, it may have an important functional role as a sphincter. The cervix constricts with uterine contractions in early labour (Olah, 1994). It is suggested that this coordinated muscular activity is important in the maintenance of cervical integrity during Braxton Hicks contractions before labour (Steer and Johnson, 1998).
Cervical ripening is predominantly an inflammatory process. Macrophages and neutrophils infiltrate the cervical tissue towards term. They produce cytokines and elastases and collagenases which digest the extracellular matrix proteins.
In practice, the cervix can be assessed by using a simple scoring system, the Bishop’s score (Table 13.1). This is particularly useful prior to induction of labour and for monitoring the changes in the cervix as the induction progresses.
If the cervix is soft, effaced and has started to dilate, induction may be implemented by artificial rupture of the membranes, which augments endogenous prostaglandin production. If the cervix is less favourable, prostaglandin E2 (PGE2) is administered into the posterior fornix of the vagina to facilitate effacement. However, PGE2 should be used with caution in multiparous women because they have a greater sensitivity to it.
The cause of these structural changes in the cervix is not clear. It is thought to be hormonally controlled. Relaxin has been shown to be important in cervical ripening in rodents and has been used clinically to promote cervical ripening in humans. However, the levels of endogenous relaxin in pregnant women seem to be highest at the beginning of the second trimester. Oestrogen affects the synthesis of connective tissue components in vitro but has limited success when used pharmacologically as an induction method. PGE2 causes cervical softening or ‘ripening’ and is produced naturally by both the cervix and the fetal membranes. PGE2 appears to act by increasing collagenolytic activity rather than by changing the composition of the ground substance, which probably precedes prostaglandin use in successful induction of labour. Following delivery, glycoproteins that bind strongly to collagen are re-formed so the rigidity of the cervix is re-established; however, it never completely regains its original form (see Chapter 14). Damage to the cervix may have long-term consequences (Box 13.1).
Box 13.1
Longitudinal fibres allow full dilatation of the cervix to be achieved without the pressure of a presenting part, for instance in the case of a transverse lie. Artificial dilatation of an unprepared cervix may damage the collagen fibres. This can result in the cervix failing to remain intact during subsequent pregnancies, resulting in habitual spontaneous abortion, usually in mid-trimester. Preoperative preparation to avoid this involves the administration of a prostaglandin derivative, which induces cervical softening and aids artificial dilatation of the cervix. This method is used prior to procedures involving exploration of the uterine cavity such as termination of pregnancy, evacuation of retained products of conception, surgical ablation of the endometrium and investigation of infertility.
Parturition in sheep has been studied in detail. Based on the assumption that mammals are likely to share similar physiological mechanisms for the onset of labour, clinical procedures derived from understanding the mechanisms of parturition in sheep were developed, both for inducing labour and for inhibiting preterm labour in humans. Progesterone is essential for pregnancy maintenance in sheep and the fetal lamb plays a crucial role in the timing of labour. Initiation of labour in sheep is driven by the maturation of the fetal brain; the effect is that progesterone falls resulting in increased uterine activity. Expression of pro-opiomelanocortin (POMC), the precursor of adrenocorticotrophic hormone (ACTH), progressively increases in the pituitary from mid-gestation as the fetal brain matures (Challis et al., 2000). Regulation of secretion of pituitary ACTH in the fetal lamb is by antidiuretic hormone (ADH, also known as vasopressin) and corticotrophin-releasing hormone (CRH). Thus, the first indicator of impending labour in sheep is a sharp rise in fetal cortisol levels due to mature secretion of ACTH from the pituitary gland and increased adrenal sensitivity to ACTH. Removal or abnormal development of the fetal lamb pituitary gland prevents the onset of labour. Infusion of ACTH, cortisone or dexamethasone (a cortisol analogue, frequently used as an anti-inflammatory drug) into the sheep fetus induces labour. Fetal stress such as hypoxia and undernutrition can stimulate preterm birth in sheep (Warnes et al., 1998) by increasing fetal HPA maturation.
The effects of raised cortisol levels are naturally efficient and both promote fetal organ maturity (functional maturation of the fetal lungs and other systems) and initiate labour. Cortisol induces 17α-hydroxylase activity in the placenta that promotes the conversion of C21 steroids to C18 steroids. Thus 17α-hydroxylase converts progesterone to oestrogen so that the progesterone:oestrogen ratio alters in favour of oestrogen. This increases prostaglandin (PGF2α) synthesis by the placenta and myometrium. The alteration in oestrogen and progesterone levels can be measured prior to the onset of labour. Exogenous oestrogen induces labour and infusion of progesterone inhibits labour in the sheep. Increasing oestrogen or decreasing progesterone levels stimulates the synthesis of PGF2α. Prostaglandins increase myometrial sensitivity to oxytocin. PGF2α is important in cervical softening and increases uterine contractility. A positive feedback mechanism, known as the Ferguson reflex, amplifies the signals. The pressure of the fetal presenting part on the cervix activates a neurohumoral reflex whereby afferent nerves from the cervix impinge on the hypothalamus and increase oxytocin release from the posterior pituitary gland. Oxytocin stimulates uterine contractions and causes further release of PGF2α from the uterus. However, initiation of labour in the human is different in a number of respects from that in the sheep. Note that pregnancy in some species, such as goats, rabbits and rodents, depends on progesterone secretion from the corpus luteum because the placenta is not the source of progesterone. Luteolysis, mediated by PGF2α, causes a fall in progesterone and initiation of parturition in these species. The fetus does not seem to play such an important role in the timing of parturition in these species as it does in sheep.
The mean length of human gestation is 39.6 weeks and the majority of births occur between 38 and 42 weeks (Steer and Johnson, 1998). Such a wide range of gestation times in normal pregnancy suggests the timing mechanism is not precise, possibly because it is affected by a number of factors including external influences. The fetus seems to maintain the pregnancy actively. Labour invariably ensues after fetal death has occurred, although it might be delayed by a few weeks (and therefore be pre-empted by mechanical removal of the products of conception). Labour occurs sooner if placental damage has occurred. The critical events permitting extrauterine survival are adequate maturation of the fetal lung and nervous system. The fetal brain may monitor this maturation and control the timing mechanism. Mothers of fetuses with brain abnormalities still progress into labour spontaneously but with a much wider range of gestation (see below). This suggests the fetal brain affects the precise timing of labour in humans, rather than controlling the exact length of gestation.
In sheep, maturation of the fetal HPA initiates parturition. Human fetal malformations such as anencephaly (no cerebrum), malformed pituitary glands or hypoplasia of the adrenal glands are associated with an increased range of gestation length (both longer and shorter) but women still undergo spontaneous labour (Steer and Johnson, 1998). In sheep, similar fetal malformations, either accidental or deliberate, result in significantly prolonged gestation which adversely affects the fetus. This implies that the fetal–adrenal axis has a supportive rather than a direct role in parturition, acting to fine tune the gestational length in humans, rather than functioning as the ‘on–off switch’ for the initiation of labour, as seen in sheep.
It is evident that the human fetal anterior pituitary gland undergoes maturational changes in the last weeks of gestation as the profile of hormonal release changes. However, there are no defined changes measurable in the maternal circulation prior to the onset of labour. The raised cortisol levels in the cord blood of infants, who have experienced a spontaneous delivery rather than an induced one, or delivery by caesarean section, are maternally derived and are secondary to maternal pain. Labour itself, whether spontaneous or induced, causes stress and therefore an increased production of cortisol, which can cross the placenta. It is possible to differentiate between cortisol of maternal origin and cortisol of fetal origin by comparing the levels in umbilical cord arterial blood with those in the cord vein. Blood in the vein flows from the placenta to the fetus so if cortisol levels are higher in the vein than in the arteries this suggests the source of cortisol is maternal.
Exogenous corticosteroids (such as dexamethasone and betamethasone) given to a pregnant woman at 24–34 weeks to promote fetal lung maturity (see Chapter 15) do not initiate parturition, although oestrogen and cortisol levels fall. Pharmacological doses of glucocorticoids introduced into the amniotic fluid increase uterine activity and induce labour; however, this effect is not observed with physiological doses. Most researchers have not been able to measure an increase in cortisol prior to the onset of labour, nor an effect on placental hormone production. Therefore, it now seems unlikely that cortisol is important in initiating labour in humans, although it has a vital role in the maturation of fetal lungs and other organs. Several factors may affect physiological responses to cortisol; these include corticosteroid-binding protein (CBP) which would influence the relative concentration of free cortisol, metabolism of cortisol to inactive cortisone by 11β-hydroxysteroid dehydrogenase and modification of corticosteroid receptor expression.
In most animal models, progesterone withdrawal is the trigger for parturition. In the sheep, fetal cortisol affects steroidogenesis so progesterone levels fall and oestrogen levels increase. This is a result of increased expression and activity of placental 17α-hydroxylase (see above). Across almost all species, the fall in plasma progesterone concentration is a common endocrine event leading to parturition. However, progesterone levels do not fall prior to labour in humans. In humans, the placenta does not express inducible 17α-hydroxylase activity and therefore cannot convert progesterone to oestrogen. However, increases in free oestriol levels have been observed in saliva of women before the onset of labour, both preterm and at term (Goodwin, 1999).
High progesterone levels inhibit myometrial activity during implantation and favour uterine quiescence throughout the pregnancy. This suppression of myometrial activity is essential to the maintenance of pregnancy, although parturition begins without a measurable decrease in maternal peripheral progesterone levels. Large doses of progesterone are relatively unsuccessful in inhibiting preterm labour in humans. However, mifepristone, the progesterone receptor antagonist previously known as RU-486, inhibits progesterone so it blocks the effect of progesterone and induces labour (Thong and Baird, 1992). Mifepristone is used clinically as an effective abortifacient. It also has anti-glucocorticoid activity and can cause ripening of the cervix and increase sensitivity to contractile prostaglandins.
It is possible in humans that there could be a functional progesterone withdrawal such as local changes in progesterone concentration or receptor binding which, even in the absence of a fall in progesterone concentration in peripheral blood, may affect myometrial activity. As the uterus enlarges during the pregnancy, the part of the uterine wall distal to the site of implantation, and therefore furthest away from the major source of progesterone production, may re-establish uterine contractions and trigger the onset of labour (Challis et al., 2000). Measurement of absolute progesterone levels may therefore be misleading as the biological effects will be altered by receptor density, levels of binding proteins or postreceptor changes. The fetal membranes and maternal decidua can both metabolize progesterone and produce cortisol. Cortisol is antagonistic to progesterone and can increase the synthesis of CRH (Karalis et al., 1996). Fetal membranes, therefore, offer a mechanism for local control of progesterone concentration. Alternative mechanisms have been suggested for the apparent lack of progesterone withdrawal prior to human parturition. These include sequestration of progesterone, another progesterone-like steroid being involved as a natural antagonist or local inactivation of progesterone, for instance progesterone is converted into an inactive metabolite that competes for receptor sites (Challis et al., 2000). For instance, before labour, the major products of the paracrine steroid hormone synthesis could be progesterone and oestrone (a weak oestrogen) and following the onset of labour the major products could be less active progesterone metabolites and biologically active oestradiol (Mitchell and Wong, 1993). It has also been suggested that in humans there is a regionalization of uterine activity and high progesterone levels are important in promoting relaxation of the lower uterine segment while contractions in the fundal area facilitate the descent of the fetus (Challis et al., 2000). Changes in progesterone receptor expression could potentially act as a functional progesterone block and thus control myometrial progesterone responsiveness (Mesiano, 2004). The altered progesterone receptor profile is postulated to modulate oestrogen effects (and hence oxytocin responses) via expression of oestrogen receptors. The human progesterone receptor exists in several isoforms produced from a single gene. PR-B is the full-length isoform which is the major mediator of progesterone effects whereas PR-A lacks the N-terminal part of the protein that contains one of the functional domains so it tends to have effects that oppose those of PR-B. There are also PR-C and two other truncated isoforms. It seems that the expression of PR-A may increase significantly before the onset of labour at term so that the ratio of the two forms changes during gestation thus affecting the response of the uterine tissue and the ability of progesterone to suppress myometrial activity (Goldman and Shalev, 2007). The increased synthesis of PR-A may be mediated by inflammatory factors and Toll-like receptors (Smith et al., 2007); this pathway may be increased in some cases of preterm labour associated with infection.
The interaction of progesterone with its receptors requires specific co-activators; these co-activators are less abundant towards the onset of labour (Smith, 2007). Progesterone metabolites may also interact with the progesterone receptors in a different way to progesterone (Mitchell and Taggart, 2009). Progesterone bioactivity could also be regulated at the post-receptor level.
Human placental production of oestrogen increases throughout labour and the rate seems to increase in the latter part of gestation. In sheep, increased oestrogen causes increased contraction-associated proteins (CAPs) and up-regulation of prostaglandin synthase and consequent increased synthesis of PGE2, promoting a positive feedback on myometrial contractility. However, spontaneous labour in humans can occur in the absence of measurable changes in oestrogen concentration. Exogenous oestrogen infusion in humans causes a transient increase in uterine activity and decreases the oxytocin threshold of the uterus but does not induce premature delivery or fetal membrane changes (Challis et al., 2000).
Synthesis of placental oestrogen depends on fetal cooperation in the provision of the precursors, and could thus potentially provide an opportunity for the human fetus to manipulate the progesterone:oestrogen ratio and uterine activity. The fetal adrenal gland in humans and higher primates is a relatively large percentage of body mass compared with the adult and has three zones: the outer adult zone produces mostly aldosterone, the unique fetal zone produces dehydroepiandrosterone sulphate (DHEAS) and the transitional zone produces mostly cortisol. This large fetal zone of the adrenal gland disappears in the neonatal period and may be regulated by hCG levels. DHEAS is the fetal adrenal C19 steroid precursor for placental oestradiol-17α and oestrone synthesis, which are implicated as having a role in labour. DHEAS is converted to oestrogens by placental sulphatase, aromatase and other enzymes (see Chapter 3). Production of DHEAS is controlled by ACTH from the anterior pituitary, which is itself regulated by hypothalamic CRH. CRH of placental origin can also stimulate production of DHEAS from the fetal zone (see below). However, women who have placental sulphatase or aromatase deficiencies and produce very little placental oestrogen can have normal pregnancy and labour (López Bernal, 2003). Thus, the changing ratio of oestrogen to progesterone may facilitate effective uterine contractions but is probably not critical for the induction of labour (Steer and Johnson, 1998).
Hypothalamic CRH controls the function of the pituitary–adrenal axis in response to stress. CRH stimulates the anterior pituitary gland to produce corticotrophin which then stimulates the cortex of the adrenal gland to release cortisol. However, even in the presence of significant stress, levels of CRH are relatively low compared to the levels reached in pregnancy. CRH is expressed abundantly in the syncytiotrophoblast cells of the human placenta (but not in non-primate placentas) and CRH-receptors are expressed in the primate placenta and myometrium. Placental CRH seems to have an important role in the initiation of parturition in higher primates and humans. CRH is synthesized by the placenta predominantly into the maternal circulation though some enters the fetal circulation (Smith, 2007). Levels of CRH steadily increase in the maternal circulation from mid-term (about 90 days before the onset of labour) until about 35 weeks when levels sharply rise and are usually particularly high in pregnancies ending with premature labour (Wolfe et al., 1990) and those complicated by pre-eclampsia (Smith and Nicholson, 2007). CRH activity is attenuated by CRH-binding protein (CRH-BP), which is synthesized by the liver, placenta and brain so most CRH is in the bound (inactive) form during pregnancy. However, towards term, when levels of CRH increase, levels of CRH-BP simultaneously fall and the capacity of the CRH-BPO is saturated so circulating levels of physiologically active, free CRH markedly increase. The maternal adrenal CRH receptors are down-regulated so the ACTH response to CRH is blunted in late pregnancy; this protects the maternal pituitary-adrenal axis from over-stimulation.
The stress hormone, cortisol, normally has a negative feedback effect to inhibit CRH secretion and thus ACTH and cortisol secretion as part of the HPA axis. The opposite situation occurs with placental CRH where cortisol has a positive feedback effect to further increase CRH release by the placenta. This positive feedback is further augmented by increased prostaglandin production (Petraglia et al., 1995), which also increases placental synthesis of CRH. Fetal stresses such as hypoxia and hypoglycaemia result in increased CRH concentrations. CRH is a vasodilator in the placental vascular bed (Clifton et al., 1994) so increased CRH should result in increased blood flow and abrogation of the fetal insult. However, if the insult persists, then CRH would increase fetal ACTH secretion and increase DHEAS production thus increasing oestrogen synthesis which leads to myometrial activation. Thus, CRH appears to be a mechanism by which a compromised fetus can precipitate labour when intrauterine life becomes unfavourable. The pathway by which CRH in the fetal circulation increases pituitary corticotrophin production which subsequently stimulates cortisol secretion by the fetal adrenal gland is important in the maturation of the fetal lungs and other organs particularly the central nervous system and gut (see Chapter 15). The maturing fetal lungs increase their production of surfactant proteins which can then enter the amniotic fluid; these surfactant proteins together with phospholipids and inflammatory cytokines are thought to stimulate inflammation in the fetal membranes and underlying myometrium and contribute to the amplification of the signals leading to labour (Smith, 2007).
It has been suggested that the ratio of placental CRH and CRH-BP acts as a placental ‘clock’ which controls the length of gestation and allows a distressed fetus to ‘wind-on’ the clock to initiate premature labour (Smith, 2007). CRH receptors can couple to different G-proteins in different tissues and at different stages of pregnancy (Karteris et al., 1998). So for most of pregnancy, CRH is coupled to the G-protein (Gαs) involved in increasing cAMP levels. It therefore has a ‘protective’ role and promotes myometrial quiescence via the generation of cAMP and cGMP, and up-regulation of nitric oxide synthase expression. Near term, the expression of CRH receptors changes to the form that couples to the other G-protein pathway (Gαq) so that CRH now activates PLC and mobilizes Ca2+ from intracellular calcium stores thus promoting increased myometrial contractility and labour (Hillhouse and Grammatopoulos, 2002). The involvement of a physiological stress pathway in the onset of labour could explain the relationship between maternal stress and reproductive failure (Nakamura et al., 2008) and the interesting possible relationship between maternal periconceptional nutritional status and the timing of parturition (MacLaughlin and McMillen, 2007). Maternal stress would stimulate the maternal pituitary-adrenal axis resulting in increased maternal cortisol production which would stimulate placental CRH release (Smith et al., 2007). Myometrial contractility is also enhanced by up-regulation of oxytocin receptor expression and cross-talk between the oxytocin and CRH receptors.
It is argued that an alternative pathway for initiation of labour is the switching off of mechanisms that cause relaxation (see above; López Bernal, 2003). Cyclic nucleotides (cAMP, etc.) promote myometrial relaxation and β2-adrenergic agonists are effective tocolytic agents (see Table 13.2). The enzyme adenylyl cyclase catalyses the production of cAMP from ATP. Coupling of receptors to adenylyl cyclase may be responsible for uterine quiescence. As progesterone inhibits activity of the phosphodiesterase which breaks down cAMP, thus terminating its action, progesterone sustains the effect of cAMP and promotes uterine relaxation during pregnancy.
Labour is an inflammatory process but the relationship between the immune system and parturition is not clear. It is clear that significant intrauterine infection can trigger parturition and cause preterm birth. Proinflammatory cytokines such as interleukin 1β (IL-1β), IL-6 and tumour necrosis factor-α (TNF-α), may have an important role in parturition (Mitchell and Taggart, 2009).
Once labour has been initiated and the myometrium is activated, there is a positive feed-forward stimulation of myometrial activity. This signal amplification is mediated by prolabour factors, known as CAPs or uterotonins. These include prostaglandins and prostanoid receptors, oxytocin and oxytocin receptors, gap junctions and ion channels. It has not been easy to discriminate between those hormones and signals that initiate labour and those that amplify the triggering signals.
Prostaglandins (PGs) of the 2-series are known to be important in the feed-forward signal amplification and progression of labour; they promote myometrial contractions, cervical dilatation and membrane rupture. Prostaglandins are present in the circulation in low concentrations and are cleared in the pulmonary circulation so they are difficult to measure as they have paracrine activity and a short half-life. Prostaglandins can be formed as a consequence of tissue trauma (including labour itself and any manipulative or tactile stimuli). In late pregnancy, prostaglandin synthesis is readily stimulated by minor local stimuli, such as coitus, vaginal examination, sweeping the membranes or amniotomy, which are associated with inducing labour. Exogenous prostaglandins can be used therapeutically to ripen the cervix and to induce uterine contractions and labour. Mid-trimester abortion can be induced by procedures, such as intra-amniotic injection of hypertonic saline, that result in the increased synthesis and release of prostaglandins. Increasing DHEAS or oestrogen concentration and decreasing progesterone concentration increase production of prostaglandins (Challis et al., 2000). An increase is myometrial expression of prostaglandins receptors, particularly the receptor of PGF2α, is implicated in the causes of some preterm labour (Olson et al., 2003).
Prostaglandins are synthesized in the fetal membranes, decidua, myometrium and cervix; levels fall abruptly following placental separation. The rate-limiting step of prostaglandin production may be important in initiating labour. Activity of phospholipase A2 (PLA2) may regulate the level of arachidonic acid, which is the precursor of prostaglandins (see Chapter 3). Increased oestrogen (or decreased progesterone) stimulates the release of PLA2 from decidual lysosomes and therefore increases free arachidonic acid and subsequent prostaglandin synthesis. Arachidonic acid can also be produced indirectly via phospholipase C activity. Alternatively, the activity of cyclooxygenase (COX) may be rate-limiting (Aitken et al., 1990). The chorion produces prostaglandin dehydrogenase (PGDH), the enzyme which inactivates prostaglandins (Smith, 2007). In late pregnancy, chorionic PGDH activity decreases so the levels of PGE2 rises.
The two most important prostaglandins in labour appear to be PGF2α and PGE2. At term, concentrations of PGE2 and PGF2α are higher in the decidua and myometrium (but these tissues are subject to tissue trauma so some authors believe raised prostaglandin levels are caused by increased uterine activity). PGE2 is involved in cervical ripening, by mediating the release of MMP, and is metabolized by the myometrium to produce PGF2α. However, women with extrauterine pregnancies go into labour at term and experience painful uterine contractions even though there are no fetal membranes in contact with the uterus.
Maintenance of human pregnancy may depend on the synthesis of PGE2 being inhibited and this inhibition of prostaglandin synthesis being attenuated at the onset of labour. Prostaglandin concentrations in the pregnant uterus are very low (about 200 times lower than at any stage in the menstrual cycle), but increase sharply in the maternal circulation from 36 weeks’ gestation probably in response to the increasing level of CRH (Hertelendy and Zakar, 2004). Arachidonic acid, the precursor, is plentiful but synthesis of prostaglandins is inhibited, even if the pregnancy is extrauterine. Progesterone or the fetus may directly or indirectly moderate synthesis or metabolism of prostaglandins. Endogenous inhibitors of prostaglandin synthesis have been identified in maternal plasma; levels fall towards the end of gestation. Inhibitors of the COX enzymes, such as aspirin and indomethacin, block prostaglandin synthesis and are used therapeutically to treat preterm labour. Prostaglandin synthesis is up-regulated by cortisol (and dexamethasone), oestradiol, CRH and the inflammatory cytokines interleukin 1β (IL-1β) and tumour necrosis factor α (TNFα; Bowen et al., 2002).
Low doses of prostaglandins, particularly PGF2α, increase myometrial responsiveness to prostaglandins and oxytocin possibly by increasing the formation of gap junctions. In vitro, PGE2 has a biphasic effect, stimulating at nanomolar concentrations and inhibiting at micromolar concentrations. It also has a dual action: when its effects are mediated by the EP1 and EP3 receptors, PGE2 increases intracellular calcium concentration, but the EP2 receptor is coupled to adenylate cyclase so PGE2 acting at this receptor decreases intracellular calcium and favours relaxation. Variations in regional prostaglandin synthesis may occur (Wilmsatt et al., 1995).
Prostacyclin (PGI2) is synthesized in the myometrium and cervix. It promotes myometrial quiescence. It has an important role in maintaining uterine blood flow in labour; it causes vasodilation of smooth muscle and inhibits platelet aggregation, potentially inhibiting thromboembolic complications. Myometrial relaxation between uterine contractions in labour prevents occlusion of the uterine vessels and hypoxia which could lead to uterine dystocia (Hertelendy and Zakar, 2004). Placental thromboxane (TxA2) has opposing effects to PGI2 and is important in the closure of the fetal ductus arteriosus and haemostasis after delivery. In pre-eclampsia, levels of prostacyclin are low and levels of thromboxane are high. This is the rationale for aspirin treatment of pre-eclampsia. Aspirin-like drugs, by inhibiting the COX enzymes and thereby prostaglandin synthesis, restore thromboxane levels in pre-eclampsia. Trials using aspirin caused a slight prolongation of gestation and diminution of uterine contractions, but also increased the risk of premature closure of the ductus arteriosus and postnatal bleeding problems in the mother.
Prostaglandins are metabolized by the enzyme PGDH which is located in the fetal membranes. Deficiency of PGDH is associated with preterm labour. Expression of PGDH is up-regulated by progesterone and IL-10 and suppressed by cortisol (and dexamethasone), oestradiol, CRH, IL-1β and TNFα. Parturition is, therefore, essentially an inflammatory process. The association between infection and preterm labour is thought to be due to the release of phospholipases from bacterial organisms (Romero et al., 2003), which cause an increase in arachidonic acid release and so prostaglandin synthesis. Decidual macrophages respond to bacterial products by releasing pro-inflammatory cytokines. Bacterial endotoxins, such as lipopolysaccharide, can either increase prostaglandin release directly or further stimulate release of cytokines which then increase prostaglandin synthesis. Lipopolysaccharide also contributes to premature rupture of the membranes.
Prostaglandins also have an important role in the establishment of a neonatal circulatory pattern (see Chapter 15). Respiratory distress syndrome is associated with high levels of PGF2α in the infant’s circulation, and patent ductus arteriosus with high PGE2 levels. PGE2 can prevent the ductus arteriosus from closing and inhibitors of prostaglandin synthesis can promote its closure. There is indirect evidence (i.e. fetal breathing movements, FBM, stop) that PGE2 increases in the fetal circulation 48–72 h before the onset of labour (Thorburn, 1992). It is possible that this is mirrored by a local increase in PGE2 concentration in uterine tissue, which initiates labour.
Oxytocin is a peptide synthesized by the hypothalamus and released from the posterior pituitary gland (see Chapter 3). A synthetic form (Oxytocinon or Syntocinon) is used extensively for induction and augmentation of human labour and can prevent postpartum bleeding or haemorrhage. Endogenous oxytocin production can also be stimulated, for instance by nipple stimulation, with a favourable outcome. However, it is not certain whether oxytocin is important in the initiation of labour. As oxytocin receptors are generally localized to the uterus, mammary glands and pituitary, oxytocin antagonists and agonists have few systemic effects. Maternal oxytocin levels are very low and do not change very much before labour. Maternal pituitary production of oxytocin dramatically increases in the first stage of labour. However, focusing on circulating levels of oxytocin may be misleading. The concentration of oxytocin receptors in the myometrium and decidua rise dramatically (by 100–200 times) during late pregnancy so the sensitivity of the uterus increases (Fuchs et al., 1984). This means the uterus can be stimulated by low concentrations of maternal oxytocin levels that previously had no effect. Therapeutic doses adequate to augment labour are very variable, which probably reflects individual differences in receptor number. If used to augment labour infusions of Oxytocinon should commenced on low dosage which is gradually increased until regular, strong contractions (around 3 in every 10 min time period) are present to ensure hyperstimulation of the myometrium is reduced. The pattern of oxytocin release changes at the onset of labour, with an increased frequency of pulses (Fuchs et al., 1991). It may be important that maternal oxytocin levels stay low during the pregnancy so the sensitivity of the uterus to oxytocin is maintained. Oxytocin antagonists, such as atosiban, have been used to inhibit uterine contractility in preterm labour (see Table 13.2) but not always effectively. Atosiban is useful in slowing down preterm labour so that steroids can be administered to facilitate fetal lung maturity however it can also cause raised blood glucose levels so should be used with caution with diabetic women (Berkman et al., 2003). Note that all tocolytic medications have side effects some of which can be extreme and potentially life-threatening (Blumenfeld and Lyell, 2009).
Get Clinical Tree app for offline access
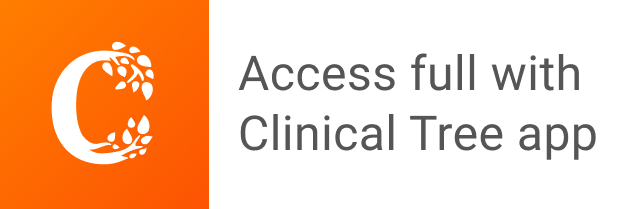