Table 18-1. MATERNAL RISK FACTORS AND POTENTIAL FETAL AND NEONATAL COMPLICATIONS | |||||||||||||||||||||||||||||||||||||||||||||||||||||||||||||||||||||||||||||||||||||||||||||||||||||||||||
---|---|---|---|---|---|---|---|---|---|---|---|---|---|---|---|---|---|---|---|---|---|---|---|---|---|---|---|---|---|---|---|---|---|---|---|---|---|---|---|---|---|---|---|---|---|---|---|---|---|---|---|---|---|---|---|---|---|---|---|---|---|---|---|---|---|---|---|---|---|---|---|---|---|---|---|---|---|---|---|---|---|---|---|---|---|---|---|---|---|---|---|---|---|---|---|---|---|---|---|---|---|---|---|---|---|---|---|
|

Table 18-2. INTRAPARTUM RISK FACTORS AND POTENTIAL FETAL AND NEONATAL COMPLICATIONS | ||||||||||||||||||||||||||||||||||||||||||||||||||||||||||||||||||||||||
---|---|---|---|---|---|---|---|---|---|---|---|---|---|---|---|---|---|---|---|---|---|---|---|---|---|---|---|---|---|---|---|---|---|---|---|---|---|---|---|---|---|---|---|---|---|---|---|---|---|---|---|---|---|---|---|---|---|---|---|---|---|---|---|---|---|---|---|---|---|---|---|---|
|
forces the remaining fetal lung fluid into pulmonary capillaries and the lymphatic circulation.
8% to 10% of fetal cardiac output (Blackburn, 2012; Steinhorn, 2011).
Smooth muscle constriction in the wall of the ductus arteriosus narrows the diameter of the ductal wall within 18 hours of birth. Permanent anatomic closure of the ductus arteriosus is usually complete within 10 to 21 days (McDaniel, 2010). Any clinical situation that causes hypoxia, with pulmonary vasoconstriction and subsequent increased PVR, potentiates right-to-left shunting (Lott, 2007). Successful transition and closure of fetal shunts creates a neonatal circulation where deoxygenated blood returns to the heart through the inferior and superior vena cava. It enters the right atrium to the right ventricle and travels through the pulmonary artery to the pulmonary vascular bed. Oxygenated blood returns through pulmonary veins to the left atrium, the left ventricle, and through the aorta to systemic circulation.
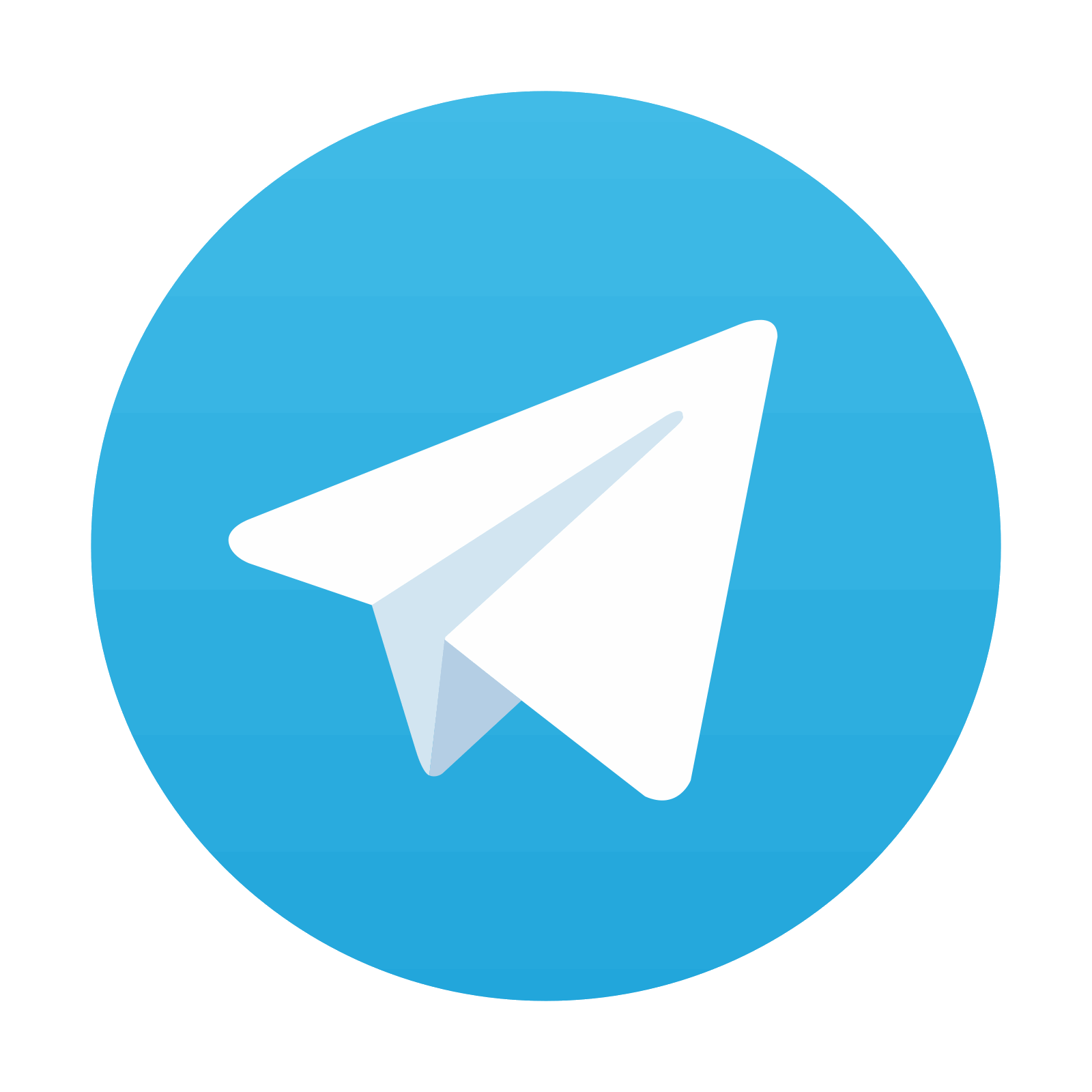
Stay updated, free articles. Join our Telegram channel
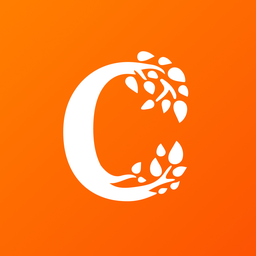
Full access? Get Clinical Tree
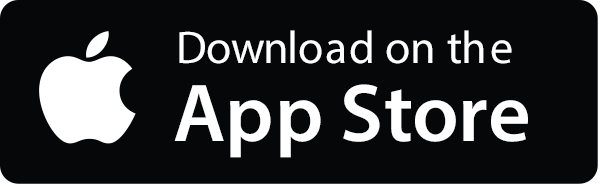
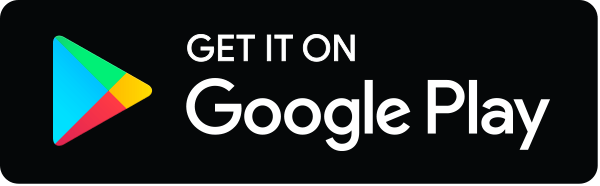
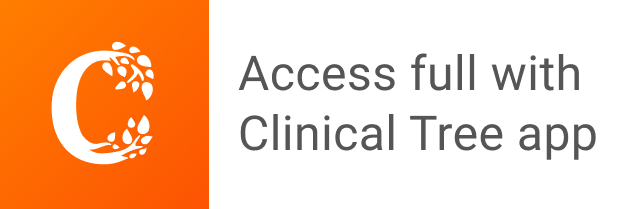