16 Neurological Assessment and Monitoring
After reading this chapter, you should be able to:
• describe the anatomy and physiology of the nervous system
• differentiate between the central and peripheral nervous systems
• describe the techniques used for neurological assessment
• identify the distinction between normal and abnormal findings
• state the determinants of intracranial pressure and describe compensatory mechanisms used to prevent large changes in intracranial pressure when there are changes in brain, blood and cerebrospinal fluid volumes
• explain the importance and process of continuous neurological assessment in the brain-injured patient
• relate the procedures of selected neurodiagnostic tests to nursing implications for patient care
Neurological Anatomy and Physiology
Components of the Nervous System
The central nervous system (CNS) consists of the spinal cord and the brain and is responsible for integrating, processing and coordinating sensory data and motor commands1 (see Figure 16.1). The CNS is linked to all parts of the body by the PNS which transmits signals to and from the CNS. The human PNS is composed of 43 pairs of spinal nerves that issue in orderly sequence from the spinal cord, and 12 pairs of cranial nerves that emerge from the base of the brain. All branch and diversify prolifically as they distribute to the tissues and organs of the body. The peripheral nerves carry input to the CNS via their sensory afferent fibres and deliver output from the CNS via the efferent fibres. Specific physiology of the CNS and PNS is discussed in detail later in the chapter. First, however, neuron cell anatomy and physiology is examined.
Neurons
Neurons are specialised cells in the nervous system; each is comprised of a dendrite, cell body (soma) and axon.2 Each neuron is a cell that uses biochemical reactions to receive, process and transmit information. Most synaptic contacts between neurons are either axodendritic (excitatory) or axosomatic (inhibitory). A neuron’s dendritic tree is connected to many neighbouring neurons and receives positive or negative charges from other neurons. The input is then passed to the soma (cell body). The primary role of the soma and the enclosed nucleus is to perform the continuous maintenance required to keep the neuron functional. Most neurons lack centrioles, important organelles involved in the organisation of the cytoskeleton and the movement of chromosomes during mitosis. As a result, typical CNS neurons cannot divide and cannot be replaced if lost to injury or disease. The fuel source for the neuron is glucose; insulin is not required for cellular uptake in the CNS.
A myelin sheath, consisting of a lipid-protein casing, covers the neuron and provides protection to the axon and speeds the transmission of impulses along nerve cells from node to node.3 (see Figure 16.2b). Myelin is not a continuous layer but has gaps called nodes of Ranvier (see Figure 16.2a).
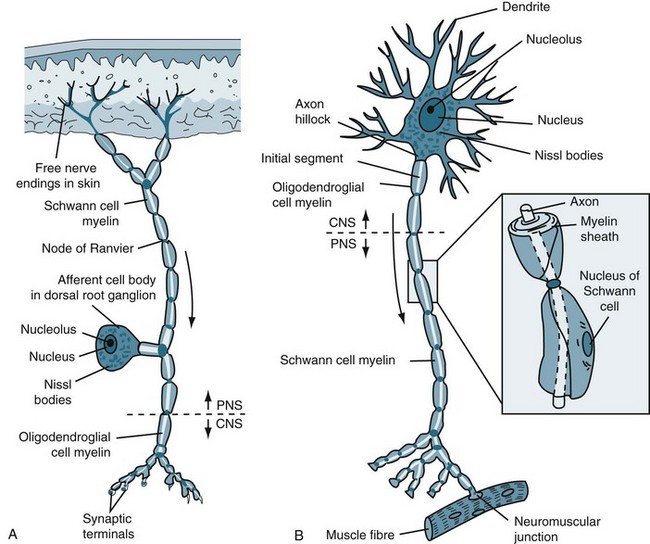
FIGURE 16.2 (A) Afferent and (B) efferent neurons, showing the soma or cell body, dendrites and axon. Arrows indicate the direction for conduction of action potentials.3
Each synaptic knob contains mitochondria, portions of the endoplasmic reticulum, and thousands of vesicles filled with neurotransmitter molecules. Breakdown products of neurotransmitter released at the synapse are reabsorbed and reassembled at the synaptic knob. The synaptic knob also receives a continuous supply of neurotransmitter synthesised in the cell body, along with enzymes and lysosomes. The movement of materials between the cell body and synaptic knobs is called axoplasmic transport. Some materials travel slowly, at rates of a few millimetres per day. This transport mechanism is known as the ‘slow stream.’ Vesicles containing neurotransmitter move much more rapidly, travelling in the ‘fast stream’ at 5–10 mm per hour which increases synaptic activity. Axoplasmic transport occurs in both directions. The flow of materials from the cell body to the synaptic knob is anterograde flow. At the same time, other substances are being transported towards the cell body in retrograde flow (’retro’ meaning backward). If debris or unusual chemicals appear in the synaptic knob, retrograde flow soon delivers them to the cell body. The arriving materials may then alter the activity of the cell by turning appropriate genes on or off. Retrograde flow is the means of transport for viruses, pathogenic bacteria, heavy metals and toxins to the CNS, with resulting disease such as tetanus, viral encephalitis and lead intoxication. Defective anterograde transport seems to be involved in certain neuropathies, including critical illness neuropathies.4
Synapses
The human brain contains at least 100 billion neurons, each with the ability to influence many other cells. Although there are many kinds of synapses within the brain, they can be divided into two general classes: electrical synapses and chemical synapses. Electrical synapses permit direct, passive flow of electrical current from one neuron to another in the form of an action potential; they are described in Table 16.1. The current flows through gap junctions, which are specialised membrane channels that connect the two cells. Chemical synapses, in contrast, enable cell-to-cell communication via the secretion of neurotransmitters; the chemical agents released by the presynaptic neurons produce secondary current flow in postsynaptic neurons by activating specific receptor molecules5 (see Figure 16.3).
TABLE 16.1 Generation of action potentials (nervous tissue)
Neurotransmitters
Chemically, there are four classes of neurotransmitters:
1. acetylcholine (ACh): the dominant neurotransmitter in the peripheral nervous system, released at neuromuscular junctions and synapses of the parasympathetic division
2. biogenic amines: serotonin, histamine, and the catecholamines dopamine and noradrenaline
3. excitatory amino acids: glutamate and aspartate, and the inhibitory amino acids gamma-aminobutyric acid (GABA), glycine and taurine
4. neuropeptides: over 50 of which are known, amino acid neurotransmitters being the most numerous.
In 2009, it was discovered that there is also more than one neurotransmitter per synapse; these are called co-transmitters. For example, neuropeptide Y (NPY) and adenosine triphosphate (ATP) are co-transmitters of noradrenaline, which are released together and mediate their function by activation of α- and β-adrenoceptors, and regulate renovascular resistance.6 Similarly, receptors are an important control point for the effectiveness of synapses. Neurotransmitters are the common denominator between the nervous, endocrine and immune systems. Many neurotransmitters are endocrine analogues and acetylcholine, the main parasympathetic neurotransmitter, interacts with immune cells such as macrophages through the anti-inflammatory cholinergic pathway.7
Neuroglia
Neuroglia are the non-neuronal cells of the nervous system and are 10–50 times more prevalent than the number of neurons.1 They are divided into macroglia (astrocytes, oligodendroglia and Schwann cells) and microglia, and are described in Table 16.2. They not only provide physical support but also respond to injury, regulate the ionic and chemical composition of the extracellular milieu, participate in the blood–brain and blood–retina barriers, form the myelin insulation of nervous pathways, guide neuronal migration during development, and exchange metabolites with neurons.8 The CNS has a greater variety of neuroglia. Unlike neurons, neuroglia continue to multiply throughout life. Because of their capacity to reproduce, most tumours of the nervous system are tumours of neuroglial tissue and not of nervous tissue itself.9
TABLE 16.2 Neuroglia, their location and role as supporting nervous tissue
Type | Location | Main Function |
---|---|---|
Astrocytes | CNS: The largest and most numerous neuroglial cells in the brain and spinal cord. | |
Ependymal cells | CNS: Line the ventricular system of the brain and central cord of the spinal canal. | |
Microglia | CNS: Located within the brain parenchyma behind the blood–brain barrier. | |
Oligodendrocytes | CNS: Spiral around an axon to form a multilayered lipoprotein coat in both the white and grey matter in the brain and spinal cord. PNS: Schwann cells are the supporting cells of the PNS. | |
Central Nervous System
The CNS is composed of the brain and spinal cord (see Figure 16.4).5 The primary purpose is to acquire, coordinate and disseminate information about the body and its environment. This section describes the anatomy and physiology of the brain and spinal cord.
Brain
The brain is divided into three regions: forebrain, midbrain and hindbrain, as described in Table 16.3. The forebrain, which consists of two hemispheres and is covered by the cerebral cortex, contains central masses of grey matter, the basal ganglia, the neural tube and the diencephalon with its adult derivatives: the thalamus and hypothalamus.1 Midbrain structures include two pairs of dorsal enlargements, the superior and inferior colliculi. The medulla, pons and midbrain compose the brainstem.1 The hindbrain includes the medulla oblongata, the pons and its dorsal outgrowth, the cerebellum.
TABLE 16.3 Organisation of the brain
Division | Description | Functions |
---|---|---|
Forebrain | ||
Cerebrum | Largest and uppermost portion of the brain. Divided into two hemispheres, each subdivided into the frontal, parietal, temporal and occipital lobes. | Cortex (outer layer) is the site of conscious thought, memory, reasoning and abstract mental functions, all localised within specific lobes. |
Diencephalon | Between the cerebrum and the brainstem. Contains the thalamus and hypothalamus. | Thalamus sorts and redirects sensory input; hypothalamus controls visceral, autonomic, endocrine and emotional function, and the pituitary gland. Contains some of the centres for coordinated parasympathetic and sympathetic stimulation, temperature regulation, appetite regulation, regulation of water balance by antidiuretic hormone (ADH), and regulation of certain rhythmic psychobiological activities (e.g. sleep). |
Brain stem | Anterior region below the cerebrum: the medulla, pons, and midbrain compose the brainstem. | Connects cerebrum and diencephalon with spinal cord. |
Midbrain | ||
Midbrain | Below the centre of the cerebrum. | Has reflex centres concerned with vision and hearing; connects cerebrum with lower portions of the brain. It contains sensory and motor pathways and serves as the centre for auditory and visual reflexes. |
Basal ganglia or corpus striatum | The mass of grey matter in the midbrain beneath the cerebral hemispheres. Borders the lateral ventricles and lies in proximity to the internal capsule. | An important role in planning and coordinating motor movements and posture. Complex neural connections link the basal ganglia to the cerebral cortex. The major effect of these structures is to inhibit unwanted muscular activity; disorders of the basal ganglia result in exaggerated, uncontrolled movements. |
Pons | Anterior to the cerebellum. | Connects cerebellum with other portions of the brain; contains motor and sensory pathways; helps to regulate respiration; axons from the cerebellum, basal ganglia, thalamus and hypothalamus; portions of the pons also control the heart, respiration and blood pressure. Cranial nerves V–VIII connect the brain in the pons. |
Hindbrain | Contains a portion of the pons, the medulla oblongata and the cerebellum. | |
Reticular activation system (RAS) | The reticular formation networks run through the brainstem core, known as the tegmentum. | Activity of the cerebral cortex is dependent on both specific sensory input and non-specific activating impulses from the RAS, and is critical to the existence of the conscious state, states of alertness and arousal. |
Medulla oblongata | Between the pons and the spinal cord. | The medulla oblongata contains motor fibres from the brain to the spinal cord and sensory fibres from the spinal cord to the brain. Most of these fibres cross at this level. Cranial nerves IX–XII connect to the brain in the medulla, which has centres for control of vital functions, such as respiration and the heart rate. |
Cerebellum | Below the posterior portion of the cerebellum. Divided into two hemispheres. | Coordinates voluntary muscles; maintains balance and muscle tone; has both excitatory and inhibitory actions. It also controls fine movement, balance, position sense and integration of sensory input. |
Nervous tissue has a high rate of metabolism. Although the brain constitutes only 3% of the body’s weight, it receives approximately 15% of the resting cardiac output and consumes 20% of its oxygen.1 Despite its substantial energy requirements, the brain can neither store oxygen nor effectively engage in anaerobic metabolism. An interruption in the blood or oxygen supply to the brain rapidly leads to clinically observable signs and symptoms. Without oxygen, brain cells continue to function for approximately 10 seconds. Glucose is virtually the sole energy substrate for the brain, and it is entirely oxidised.10 The brain can be seen as an almost exclusive glucose-processing machine, producing water (H2O) and carbon dioxide (CO2). Glucose also provides the carbon backbone for regeneration of the neuronal pool of glutamate. This process results from close astrocyte–neuron cooperation.11
Cerebral cortex
The forebrain contains the cerebral cortex and the subcortical structures rostral (sideways) to the diencephalon. The cortex, or outermost surface of the cerebrum, makes up about 80% of the human brain. The cerebral cortex varies in thickness from 2 mm to 4 mm, being thinnest in the primary sensory areas and thickest in the motor and association areas. It contains the cell bodies and dendrites of neurons or grey matter which receive, integrate, store and transmit information. Conscious deliberation and voluntary actions also arise from the cerebral cortex. White matter lies beneath the cerebral cortex and is composed of myelinated nerve fibres. The cortex is involved in the processing of both sensory information from the body and the delivery of motor commands. These occur in specific areas of the brain and can be mapped. Topographically, the cerebral cortex is divided into areas of specialised functions, including the primary sensory areas for vision (occipital cortex), hearing (temporal cortex), somatic sensation (postcentral gyrus), and primary motor area (precentral gyrus). As shown in Figure 16.5,1 these well-defined areas comprise only a small fraction of the surface of the cerebral cortex.
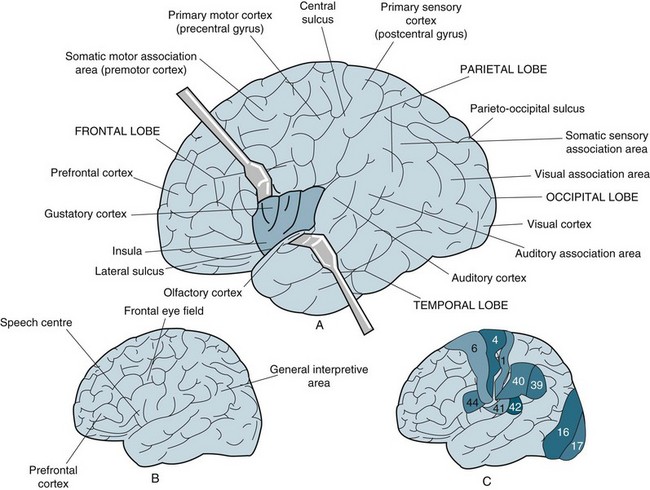
FIGURE 16.5 (A) Major anatomical landmarks on the surface of the left cerebral hemisphere. The lateral sulcus has been pulled apart to expose the insula. (B) The left hemisphere generally contains the general interpretive area and the speech centre. The prefrontal cortex of each hemisphere is involved with conscious intellectual functions. (C) Regions of the cerebral cortex as determined by histological analysis. Several of the 47 regions described by Brodmann are shown for comparison with the results of functional mapping.1
The majority of the remaining cortical area is known as the association cortex, where the processing of extensive and sophisticated neural information is performed.12 The association areas are also sites of long-term memory, and they control human functions such as language acquisition, speech, musical ability, mathematical ability, complex motor skills, abstract thought, symbolic thought, and other cognitive functions. Association areas interconnect and integrate information from the primary sensory and motor areas via intra-hemispheric connections. The parietal–temporal–occipital association cortex integrates neural information contributed by visual, auditory, and somatic sensory experiences. The prefrontal association cortex is extremely important as the coordinator of emotionally motivated behaviours, by virtue of its connections with the limbic system. In addition, the prefrontal cortex receives neural input from the other association areas and regulates motivated behaviours by direct input to the premotor area, which serves as the association area of the motor cortex. Sensory and motor functions are controlled by cortical structures in the contralateral hemisphere. Particular cognitive functions or components of these functions may be lateralised to one side of the brain.
The cerebral cortex receives sensory information from many different sensory organs and processes the information. The two hemispheres receive the information from the opposite sides of the body. Sensory information is relayed to the cortex by the thalamus. Parts of the cortex that receive this information are called primary sensory areas and cross at various points in the sensory pathway, because the cerebral cortex operates on a contralateral basis.13 The discriminative touch system crosses high, in the medulla. The pain system crosses low, in the spinal cord. The proprioceptive sensory system that guards balance and position goes to the cerebellum, which works ipsilaterally and therefore doesn’t cross. Almost every region of the body is represented by a corresponding region in both the primary motor cortex and the somatic sensory cortex.14
The homunculus (see Figure 16.6) visualises the connection between different areas of the body and areas in brain hemispheres.15 The body on the right side is the motor homunculus and on the left the sensory homunculus. Representations of parts of the body that exhibit fine motor control and sensory capabilities occupy a greater amount of space than those that exhibit less precise motor or sensory functions.
Basal ganglia and cerebellum
The basal ganglia, consisting of the caudate, putamen, globus pallidus, substantia nigra, subthalamic nucleus, and related nuclei in the brainstem, play an important role in movement, as evidenced by the hypokinetic/rigid and hyperkinetic disorders seen with lesions of various components. However, their role in the initiation and control of movement cannot be isolated from the motor activities of the cortex and brainstem centres discussed previously. Procedural memories for motor and other unconscious skills depend on the integrity of the premotor cortex, basal ganglia and cerebellum.16 The cerebellum plays a more obvious role in coordinating movements by giving feedback to the motor cortex, as well as by providing important influences on eye movements through brainstem connections, and on postural activity through projections down the spinal cord.
Brainstem
The brainstem is composed of the midbrain, the pons and the medulla oblongata.1 These structures connect the cerebrum and diencephalon with the spinal cord. Brainstem centres are organised into medial, lateral and aminergic systems. Collectively, these integrate vestibular, visual and somatosensory inputs for the control of eye movements and, through projections to the spinal cord, provide for postural adjustments. For example, these centres keep the images on matching regions of the retinas when the head moves by causing conjugate eye movements in the opposite direction to which the head is turned. This is the basis for the ‘doll’s eyes’ test in neurological assessment, in which the head is rapidly turned and the eyes move conjugately in the opposite direction, demonstrating the integrity of much of the brainstem. The sequence of sleep states is governed by a group of brainstem nuclei that project widely throughout the brain and spinal cord.17
The midbrain, inferior to the centre of the cerebrum, forms the superior part of the brainstem. It contains the reticular formation (which collects input from higher brain centres and passes it on to motor neurons), the substantia nigra (which regulates body movements; damage to the substantia nigra causes Parkinson’s disease) and the ventral tegmental area (which contains dopamine-releasing neurons that are activated by nicotinic acetylcholine receptors).18 White matter at the anterior of the midbrain conducts impulses between the higher centres of the cerebrum and the lower centres of the pons, medulla, cerebellum and spinal cord. The midbrain contains the autonomic reflex centres for pupillary accommodations to light, which constrict the pupil and accommodate the lens. The fibres travel in cranial nerve III, so damage to that nerve will also produce a dilated pupil. It also contains the ventral tegmental area, packed with dopamine-releasing neurons that synapse deep within the forebrain and seem to be involved in pleasure: amphetamines and cocaine bind to the same receptors that it activates, and this may account at least in part for their addictive qualities.
The medulla oblongata lies between the pons and the spinal cord and looks like a swollen tip to the spinal cord. Running down the ventral aspect of the medulla are the pyramids, which contain corticospinal fibres. The function of the medulla oblongata is to control automatic functions (e.g. breathing and heart rate) and to relay nerve messages from the brain to the spinal cord. Processing of interaural time differences for sound localisation occurs in the olivary nuclei. The neurons controlling breathing have mu (µ) receptors, the receptors to which opiates bind. This accounts for the suppressive effect of opiates on breathing. Impairment of any of the vital functions or reflexes involving these cranial nerves suggests medullary damage.19
The pons varolii is the part of the brainstem that lies between the medulla oblongata and the mesencephalon. It contains pneumotaxic and apneustic respiratory centres and fibre tracts connecting higher and lower centres, including the cerebellum. The pons seems to serve as a relay station, carrying signals from various parts of the cerebral cortex to the cerebellum. Nerve impulses coming from the eyes, ears and touch receptors are sent on to the cerebellum. The pons also participates in the reflexes that regulate breathing. Table 16.4 contains a description of the cranial nerves including their type of tract, their function and location of origin.
Hypothalamus and limbic system
The hypothalamus, the cingulate gyrus of the cortex, the amygdala and hippocampus in the temporal lobes, and the septum and interconnecting nerve fibre tracts among these areas comprise the limbic system. The hypothalamus and limbic systems, which are closely linked to homeostasis, act to regulate endocrine secretion and the autonomic nervous system, and to influence behaviour through emotions and drives.1 The hypothalamus integrates information from the forebrain, brainstem, spinal cord and various endocrine systems. This area of the brain also contains some of the centres for coordinated parasympathetic and sympathetic stimulation, as well as those for temperature regulation, appetite regulation, regulation of water balance by antidiuretic hormone (ADH), and regulation of certain rhythmic psychobiological activities (e.g. sleep). The release of stored serotonin from axon terminals in the diencephalon, medulla, thalamus, and a small forebrain area (DMTF), results in inactivation of the RAS and activation of the DMTF. DMTF activity results in the four stages of sleep. The hypothalamus contains a plethora of neurotransmitters. These are found in the terminals of axons that originate from neurons outside the hypothalamus, but most are synthesised within the hypothalamus itself. The list of putative neurotransmitters includes the ‘classic’ transmitters ACh, GABA, glutamate, serotonin, dopamine and noradrenaline, as well as literally dozens of peptides that have been identified in recent years.20
Protection and Support of the Brain
Cerebral Spinal Fluid
Cerebral spinal fluid (CSF) is an ultrafiltrate of blood plasma composed of 99% water with other constituents, making it close to the composition of the brain extracellular fluid.1 Approximately 500 mL CSF is secreted each day, but only approximately 150 mL is in the ventricular system at any one time, meaning that the CSF is continuously being absorbed. The CSF produced in the ventricles must flow through the interventricular foramen, the third ventricle, the cerebral aqueduct and the fourth ventricle to exit from the neural tube.21 Three openings, or foramina, allow the CSF to pass into the subarachnoid space (see Figure 16.7).1 Approximately 30% of the CSF passes down into the subarachnoid space that surrounds the spinal cord, mainly on its dorsal surface, and moves back up to the cranial cavity along its ventral surface. Reabsorption of CSF into the vascular system occurs, through a pressure gradient. The normal CSF pressure is approximately 10 mmHg in the lateral recumbent position, although it may be as low as 5 mmHg or as high as 15 mmHg in healthy persons. The microstructure of the arachnoid villi is such that if the CSF pressure falls below approximately 3 mmHg the passageways collapse, and reverse flow is blocked. Arachnoid villi function as one-way valves, permitting CSF outflow into the blood but not allowing blood to pass into the arachnoid spaces. The pressure in the CSF manifests as normal ICP.
Blood–Brain–Cerebral Spinal Fluid Barrier
The CNS is richly supplied with blood vessels that bring oxygen and nutrients to the cells there. However, many substances cannot easily be exchanged between blood and brain because the endothelial cells of the vessels and the astrocytes of the CNS form extremely tight junctions, collectively referred to as the blood–brain barrier (BBB).1 In particular, small non-charged, lipid-soluble molecules can cross the BBB with ease. Experimental and clinical evidence suggests that the BBB maintains the chemical environment for neuronal function and protects the brain from harmful substances.22 Substances in the blood that gain rapid entry to the brain include glucose, the important source of energy, certain ions that maintain a proper medium for electrical activity, and oxygen for cellular respiration. Small fat-soluble molecules, like ethanol, pass through the BBB. Some water-soluble molecules pass into the brain carried by special proteins in the plasma membrane of the endothelial cells. Excluded molecules include proteins, toxins, most antibiotics, and monoamines (e.g. neurotransmitters). Some of these unwanted molecules are actively transported out of the endothelial cells. When injured (by force or infection or oxidative processes), the permeability of the BBB is disrupted, allowing a proliferation of various chemicals and molecules – even bacteria – into the brain parenchyma, with at times devastating consequences.
Cerebral Circulation
The brain must maintain a constant flow of blood in order for brain activity to occur. The arterial blood flow to the brain consists of approximately 20% of the cardiac output (see Figure 16.8).5 Normal cerebral blood flow is 750 mL/min. The brain autoregulates blood flow over a wide range of blood pressure by vasodilation or vasoconstriction of the arteries.1 In response to decreased arterial flow, the circle of Willis can act as a protective mechanism by shunting blood from one side to the other or from the anterior to posterior portions of the brain. This compensatory mechanism delays neurological deterioration in patients.
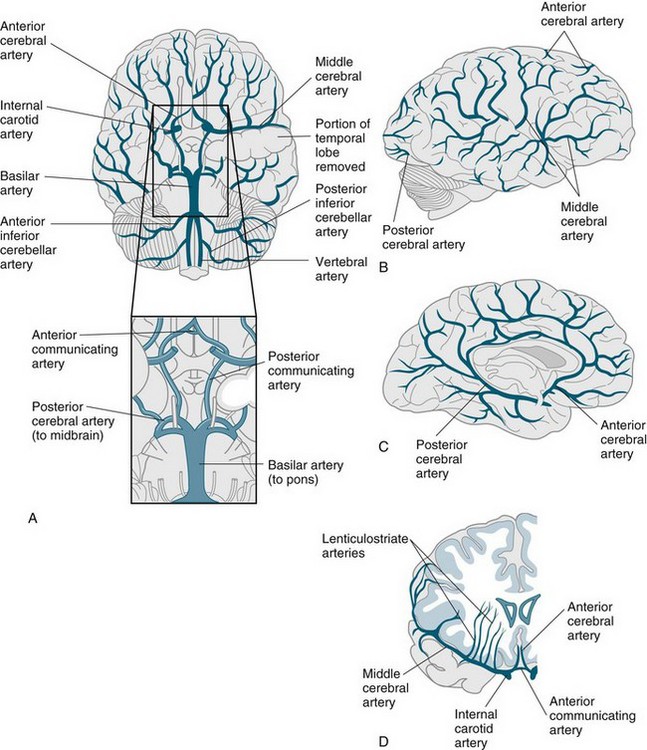
FIGURE 16.8 The major arteries of the brain: (A) ventral view: the enlargement of the boxed area showing the circle of Willis; (B) lateral and (C) midsagittal views showing anterior, middle and posterior cerebral arteries; (D) idealised frontal section showing course of middle cerebral artery.82
The cerebral veins drain into large venous sinuses and then into the right and left internal jugular veins (see Figure 16.9).23 The venous sinuses are found within the folds of the dura mater. The veins and sinuses of the brain do not have valves, so the blood flows freely by gravity.1 The face and scalp veins can flow into the brain venous sinuses; therefore, infection can easily be spread into the dural venous sinuses and then enter the brain. Patient position can prevent or promote venous drainage from the brain. Head turning and tilting may kink the jugular vein and decrease or stop venous flow from the brain, which will then raise the pressure inside the cranial vault.
Cerebral blood flow (CBF) is the cerebral perfusion pressure (CPP) divided by cerebrovascular resistance (CVR). CVR is the amount of resistance created by the cerebral vessels, and it is controlled by the autoregulatory mechanisms of the brain. Specifically, vasoconstriction (and vasospasm) will increase CVR, and vasodilation will decrease CVR.1 It is influenced by the inflow pressure (systole), outflow pressure (venous pressure), cross-sectional diameter of cerebral blood vessels, and intracranial pressure (ICP).1 CVR is similar to systemic vascular resistance; but, due to the lack of valves in the venous system of the brain, cerebral venous pressure also influences the CVR. An important characteristic of the cerebral circulation is its ability to autoregulate, that is, the ability to maintain constant cerebral blood flow despite variations in perfusion pressure (see Table 16.5). This is important in protecting the brain from both ischaemia during hypotension and haemorrhage during hypertension. CBF is affected by extrinsic and intrinsic factors.1 Extrinsic factors include systemic blood pressure, cardiac output, blood viscosity and vascular tone. The body responds to these demands with changes in blood flow. Aerobic metabolism is critically dependent on oxygen in order to process glucose for normal energy production, and the brain does not store energy. Therefore, without a constant source of oxygen and energy, its supply from CBF can be exhausted within 3 minutes. Intrinsic factors include PaCO2 (pH), PaO2 and ICP. The vessels dilate with increases in PaCO2 (hypercarbia) or low pH (acidosis) and with decreases in PaO2 (hypoxia). This vasodilation increases CBF. The vessels constrict with decreases in PaCO2 or high pH and with increases in local PaO2.1 This vasoconstriction will decrease the CBF. In addition, intrinsic factors can change the extrinsic factors by altering the metabolic mechanisms. These changes can lead to an alteration in the CBF. For example, there can be a change from aerobic to anaerobic metabolism, which increases the concentrations of other end-products such as lactic acid, pyruvic acid and carbonic acid, which causes a localised acidosis. These end-products result in a high pH which will cause an increase in CBF. Other factors that can affect CBF include pharmacological agents (anaesthetic agents and some antihypertensive agents), rapid-eye-movement sleep, arousal, pain, seizures, elevations in body temperature, and cerebral trauma.
TABLE 16.5 Changes in cerebrovascular and cerebrometabolic parameters when various cerebral variables are reduced with and without intact autoregulation
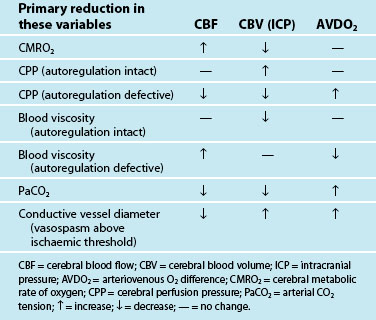
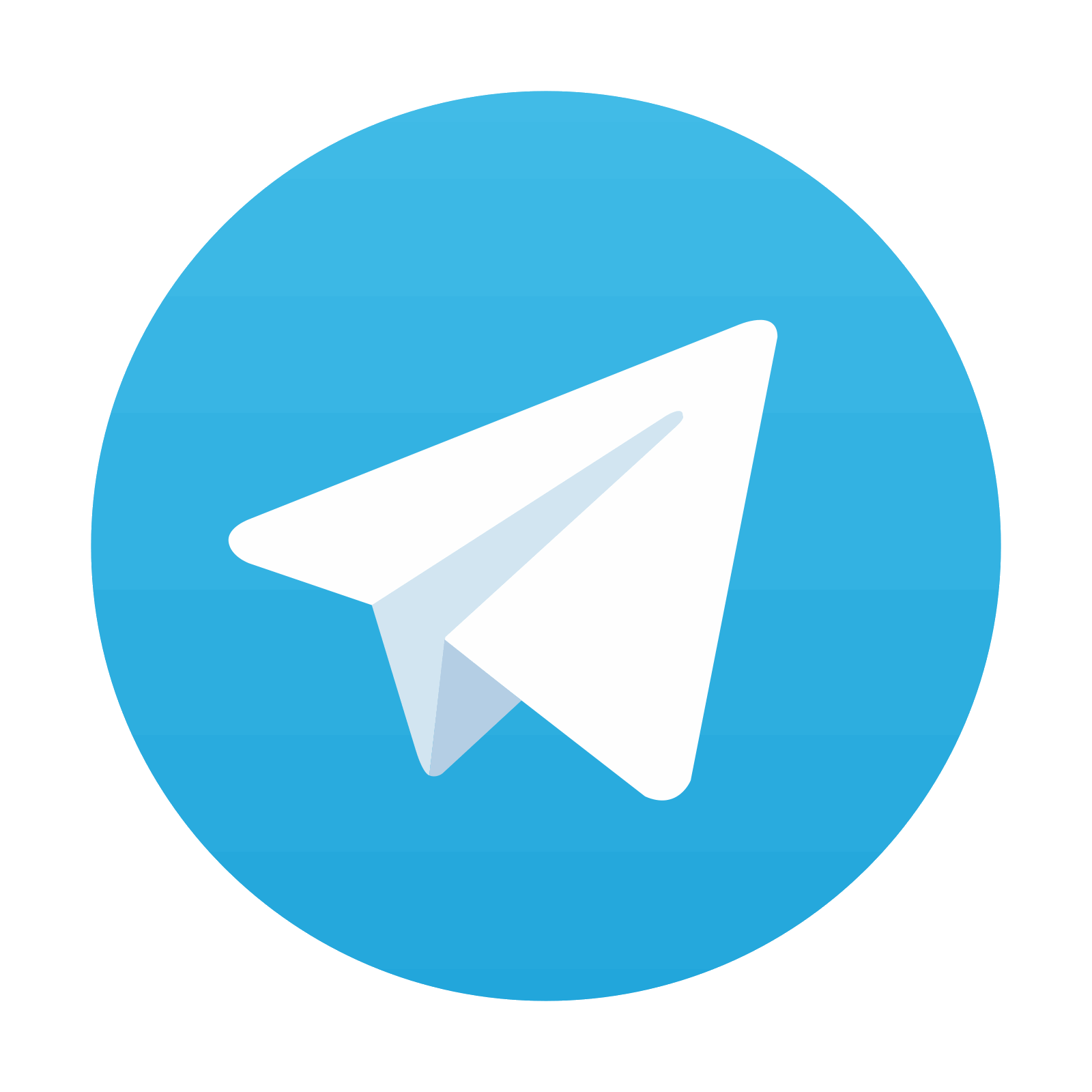
Stay updated, free articles. Join our Telegram channel
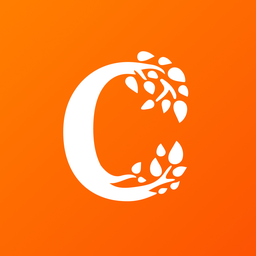
Full access? Get Clinical Tree
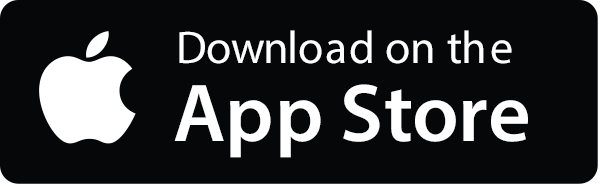
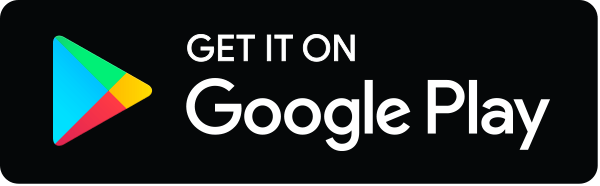