Neurologic Anatomy and Physiology
The nervous system is the “executive suite” of the human body. It directs all other systems and provides the unique ability for thought, emotion, understanding of complex information, and integration of numerous stimuli. As the recipient of all sensory information for analysis, the nervous system generates intellectual and motor responses aimed at maintaining the integrity of life structures. Critical care nurses must attain a basic understanding of the anatomy and physiology of this complex system because it serves as the basis for innervation and proper functioning of all other systems. This chapter reviews the anatomic divisions and functions of the central nervous system (CNS), including the cellular microstructure, protective encasement, networked functions, and mechanisms aimed at maintenance of structural and physiologic integrity. The cranial nerves, a component of the peripheral nervous system (PNS), are presented in tabular form.
Divisions of the Nervous System
The nervous system is the most highly organized system of the body, with all of its parts functioning as an inseparable unit. This system is usually classified by anatomic function.
Physiologic Divisions
The somatic, or voluntary, nervous system is composed of fibers that connect the CNS with structures of the skeletal muscles and the skin. The autonomic, or involuntary, nervous system is composed of fibers that connect the CNS with smooth muscle, cardiac muscle, internal organs, and glands. It includes sympathetic and parasympathetic branches.1,2
Most activities of the nervous system originate from sensory receptors such as visual, auditory, or tactile receptors. This sensory information is transmitted to the CNS by afferent fibers (sensory fibers). Efferent fibers (motor fibers) transmit the CNS response to the periphery to produce a motor response such as contraction of skeletal muscles, contraction of the smooth muscles of organs, or secretion by endocrine glands. To better understand the macrostructure and functions of the nervous system, it helps to study the microstructure, or the cellular level.1–4
Microstructure of the Nervous System
Two types of cells make up the nervous system: 1) neurons and 2) neuroglia. Neurons are the cells primarily charged with the functional work of the nervous system, including receipt of information, integration, and transmission or conduction of nerve impulses to recipient cells. Neuroglial cells serve as the support infrastructure of the nervous system, providing protection and a structural foundation for neurons and participating in neuronal repair.1–4
Neuroglia
In the nervous system, 6 to 10 times more neuroglial cells exist than do neurons. Neuroglial cells consist of four types: 1) astroglia (astrocytes), 2) oligodendroglia, 3) ependyma, and 4) microglia (Fig. 22-1). These cells provide the neuron with structural support, nourishment, and protection (Table 22-1).1–4 They retain the ability to replicate, but they can replicate abnormally and therefore are the primary source of CNS neoplasms.3,4
TABLE 22-1
CELL TYPE | FUNCTION |
Astroglia (astrocyte) | Supplies nutrients to neuron structure and to support framework for neurons and capillaries; forms part of the blood–brain barrier |
Oligodendroglia | Forms the myelin sheath in the central nervous system |
Ependyma | Lines the ventricular system; forms the choroid plexus, which produces cerebrospinal fluid |
Microglia | Occurs mainly in the white matter; phagocytizes waste products from injured neurons |
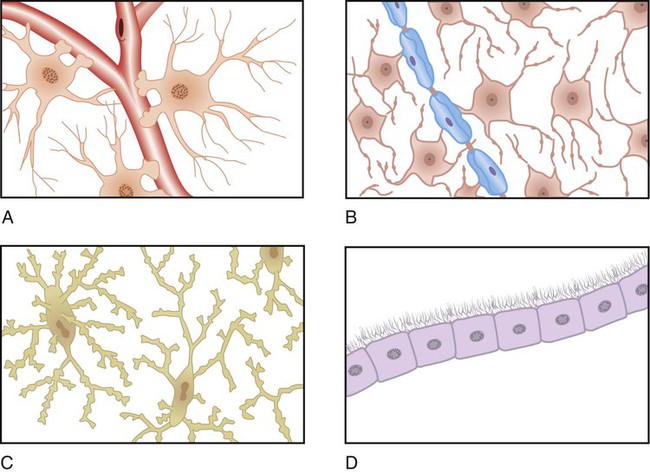
Neurons
Neurons are the basic functional unit within the CNS, and they are charged with the highly specialized task of data integration and signal transmission. The CNS is made up of more than 10 billion neurons.1,2 The cellular appearance of neurons varies, but each cell contains three basic components: 1) the cell body, 2) dendrites, and 3) an axon (Fig. 22-2). Neurons are structurally classified as unipolar, a cell body with one process that divides into a central branch (one axon) and a peripheral branch (one dendrite); as bipolar, a cell body with two processes (one axon and one dendrite); or multipolar, a cell body with one axon and several dendrites. The cell body (soma) controls the metabolic activity of the neuron and contains the organelles such as the nucleus, mitochondria, endoplasmic reticulum, Golgi apparatus, and liposomes, which are necessary for cellular metabolism and maintenance.1–3 Compared with other body cells, the neuron’s protein-embedded membrane with its phospholipid bilayer is unique, consisting of specialized pores that work as ion-specific channels or pumps to promote passage of ions through an otherwise impermeable plasma membrane.4
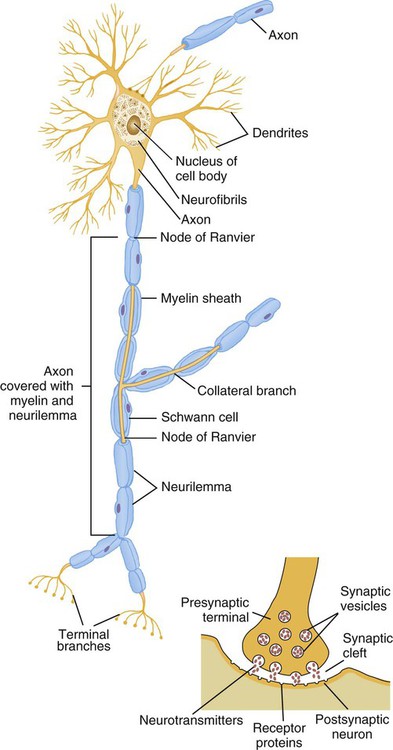
The neuronal cell body is the life support unit of the neuron. The metabolic demands of these specialized units necessitate uninterrupted perfusion with glucose and oxygen to maintain neuronal life and optimal functioning. Until recently, it was believed that CNS neuronal repair was impossible, but research has validated that neurons are more plastic than was previously thought, although rates of repair (plasticity) or restoration of neuronal function are driven by factors that remain largely unknown.5–7 Within the brain and the spinal cord, neuronal cell bodies make up regions of gray matter. Outside the CNS, ganglia are cell bodies within the PNS that reside near and work closely with CNS neurons.1–4
Dendrites form the receptive component of the neuron; they are branched fibers extending only a short distance from the cell body. Each neuron may have several dendrites, which function as impulse receivers for the cell body.1,2,7 The axon is the part of the neuron concerned with transmission of impulses away from the cell body to other neurons, muscle cells, endocrine glands, or some other effector organ.8 Neurons contain only one axon, whose length may be microscopic or, in some cases, may extend up to 4 feet. Some axons are protected by a myelin sheath, a white phospholipid complex laid down by Schwann cells in the PNS and by oligodendroglia in the CNS. Myelin sheathes protect neuronal axons and provide insulation for the conduction of nerve impulses.1,2,9 Fibers enclosed in the sheath are called myelinated fibers; those not enclosed are called unmyelinated fibers. The white matter of the CNS is composed of myelinated fiber tracts.1,2
Myelinated fibers use a process called saltatory conduction to support rapid axonal transmission of nerve impulses.3,4,9 Structurally, axons participating in this form of impulse transmission are laid out with a noncontinuous myelin cover, interrupted with 2 micrometer bare segments called the nodes of Ranvier. These nodes are packed with sodium channels, making them extremely sensitive to membrane depolarization. Because segments of the axon covered by myelin are impervious to sodium influx, impulse transmission is pulled down the length of the axon to the next node of Ranvier. Saltatory conduction increases transmission velocity up to 100-fold, allowing transmission at rates as high as 120 meters per second (m/s).1,4
Neuronal function is driven by depolarization–repolarization cycles, similar to that described for cardiac physiology (see Chapter 12), but what makes the nervous system so exceptional is its ability to undergo the depolarization–repolarization cycle up to 1000 times per second to ensure optimal receipt, integration, and transmission of information throughout the body.3 The movement of ions across the neuronal membrane generates electrical action potentials (Fig. 22-3). Neuronal resting membrane potential (RMP) is −65 millivolts (mV), approximating the equilibrium potential for potassium; on depolarization, sodium channels open, shifting the equilibrium potential in the positive direction.1,3,4
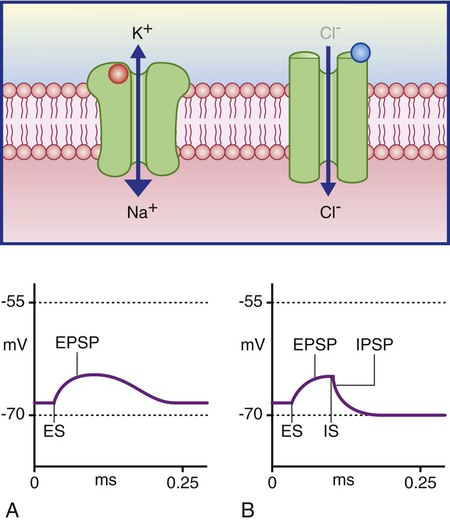
Mechanisms for ionic movement involve two types of neuronal channels: 1) voltage-gated and 2) ligand-gated. Many pharmaceutical and therapeutic agents currently in use or undergoing testing manipulate these ionic transport mechanisms. Voltage-gated channels become activated with changes in transmembrane electrical potential, promoting sodium and calcium influx and potassium efflux. These channels are the primary drivers of cellular action potentials.1,2 Ligand-gated channels are primarily concerned with mitigating the response of a postsynaptic neuron to synapse and are discussed in more detail later.1,2
Action potentials begin with the influx of sodium, producing a focal zone of membrane depolarization at some level between −55 mV to −35 mV. After this critical threshold is reached, a large number of sodium channels open, resulting in fast and massive localized depolarization of the plasma membrane. Rapid sodium influx (upstroke phase) increases the membrane potential to between 70 mV and 90 mV. As the membrane potential changes locally, it stimulates adjoining regions in the neuron to begin depolarization in a self-propagating fashion until depolarization is complete. Within a millisecond of opening, sodium channels close and become inactive.1–4
Depolarization causes potassium channels to open, allowing this ion to flow out into the extracellular space, thereby promoting the onset of repolarization. Potassium efflux triggers the cell membrane to return to a potassium equilibrium potential of approximately −75 mV, allowing potassium to re-enter the cell but maintaining greater polarity than RMP to hold the cell refractory to another depolarization stimulus. Cellular pumps that depend on a steady supply of adenosine triphosphate (ATP) are also activated to remove sodium and restore the −65 mV RMP, allowing the cycle to begin anew.1–4
After an action potential reaches the axon terminal, it initiates a cascade of events that promote interneuronal communication, or synapse. Two types of synapse exist: 1) electrical and 2) chemical. In an electrical synapse, gap junctions made up of narrow (3.5-nm) bridges allow cytoplasm and intracellular metabolites to pass in an essentially continuous fashion between neurons, facilitating impulse conduction from one neuron to the next.1,2,4 In a chemical synapse, which is involved in most synaptic events, no physical bridge exists between neurons. Instead, a large synaptic cleft of 20 to 40 nanometers (nm) prevents direct action potential transmission from one neuron to another. When the wave of depolarization reaches the presynaptic terminal, it signals the release of neurotransmitters into the synaptic cleft.1,2
The two classifications of neurotransmitters are: 1) small-molecule transmitters and 2) neuroactive peptides. Examples of small-molecule transmitters include acetylcholine, dopamine, norepinephrine, epinephrine, serotonin, histamine, gamma-aminobutyric acid (GABA), glycine, and glutamate. Neuroactive peptides include such substances as pituitary peptides, hypothalamic-releasing hormones, and neurohypophyseal hormones. This chapter is primarily concerned with the small-molecule transmitters, which are stored in vesicles within the axon terminal and released into the synapse through a process called exocytosis. Exocytosis is stimulated by arrival of the action potential in the axon terminal and results in release of neurotransmitters into the synaptic cleft, where these molecules rapidly diffuse to interact with postsynaptic receptors.1,2,4
Ligand-gated channels are activated by binding of ligand agonists to receptors on the postsynaptic neuron. Ions passing through ligand-gated channels promote an excitatory or inhibitory response within postsynaptic neurons. Examples of excitatory ligand-gated channel receptors include the inotropic glutamate receptors (N-methyl-D-aspartate [NMDA], alpha-amino-3-hydroxyl-5-methyl-4-isoxazolepropionic acid [AMPA], and kainite), which are primarily concerned with gating sodium, potassium, and calcium ions. Examples of inhibitory ligand-gated channel receptors include GABA receptors, glycine receptors, and nicotinic acetylcholine receptors. Most GABA receptors are found in the brain, where GABA serves as the primary inhibitory neurotransmitter, whereas glycine serves as the spinal cord’s primary postsynaptic inhibitory neurotransmitter. GABA and glycine channels gate chloride ions, which inhibit by promoting repolarization to the chloride equilibrium potential (−60 mV) and by short-circuiting incoming excitatory potentials by gating anions and clamping the membrane shut to excitatory cations (shunting). In other words, when inhibitor neurotransmitters are released, the neuron’s internal charge becomes more negative, and the resistance to depolarization is increased.1
Metabotropic receptors contribute to impulse transmission, promoting sustained effects of postsynaptic excitation or inhibition. Examples of metabotropic receptors include catecholamine receptors, neuropeptide receptors, and muscarinic receptors.1,4
Termination of the synapse reaction is most commonly accomplished through reuptake, in which transporter proteins embedded in neuron and glial cell membranes direct neurotransmitter molecules within the cleft to move back to the intracellular compartment for vesicle repackaging. Neurotransmitters may also go through enzyme degradation, with their component parts taken up for further neurotransmitter synthesis and storage. Ultimately, remaining neurotransmitter diffuses away from the synaptic cleft.1,2,4
The response in the postsynaptic neuron to synapse is an excitatory or inhibitory potential. Membrane potentials are not strong enough by themselves to generate a complete action potential within the postsynaptic neuron, but they are, instead, summarized or integrated by the neuronal cell body in the process of information transmission.9 When the postsynaptic neuron is bombarded with excitatory potentials, they may combine (summation) to become capable of stimulating an action potential.1–4
Examples of disease-induced or chemically-induced mechanisms that alter neuronal transmission are provided in Figure 22-4.
Central Nervous System
The CNS consists of the brain and the spinal cord. Serving as the control unit for all body systems, the remarkably delicate CNS requires significant protection to preserve normal function. This section addresses the anatomy and physiology of the brain and the spinal cord, supporting the critical care nurse’s understanding of pathophysiologic changes that contribute to clinical examination findings.
Cranial Protective Mechanisms
Bony Structures
The outermost protective measures underneath the integument are the bony structures that encase the CNS. The skull, or cranium, surrounds the brain and is composed of eight flat, irregular bones fused at sutures during early childhood (Fig. 22-5).1,2 The skull protects the brain from direct force and superficial trauma, although excessive force may fracture the skull, destroying this protective mechanism and driving bony fragments into fragile brain tissue.10
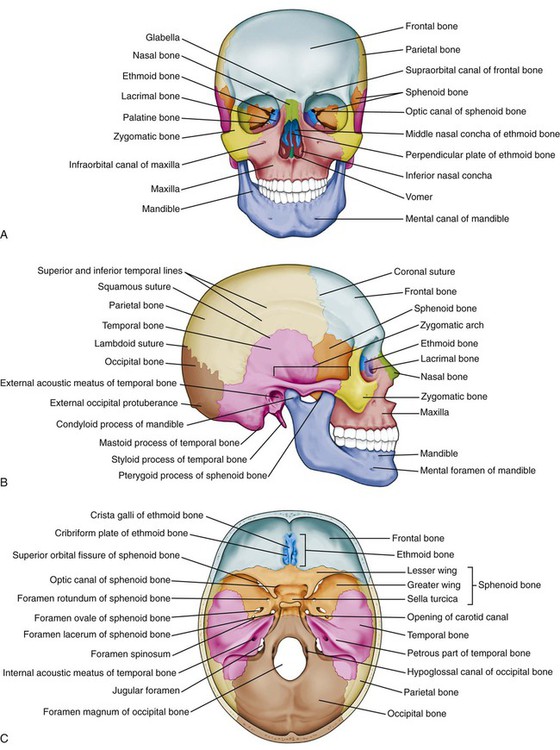
If the skull is seen from the inside, the superior surfaces form a smooth inner wall, whereas the basilar skull contains ridges and folds with sharp edges.1,2 Traumatic impact to the head often results in fracture of the basilar skull as a result of gravitational forces that displace energy in a downward fashion toward the skull base.
The cranium is a solid, nonexpanding bony vault with only one large opening at the base called the foramen magnum, through which the brainstem projects and connects to the spinal cord. Several other very small openings in the base of the skull allow entrance and exit of blood vessels and nerve fibers.1,2
Meninges
Directly beneath the skull lie the meninges, which form another source of protection for the CNS. The meninges consist of three layers: 1) the dura mater, 2) the arachnoid mater, and 3) the pia mater (Fig. 22-6).1,2,11
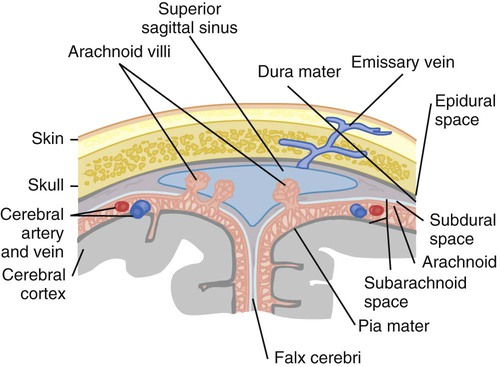
Dura Mater.
The outermost layer of meninges directly beneath the skull is the dura mater. Dura is the Latin term for “tough,” and true to its name, this layer is made up of fibrous tissue that is double-folded to support the CNS, nerves, and vascular structures.1,3 Within the dura mater’s double layers lie venous sinuses that collect blood from intracranial and meningeal veins for drainage into the internal jugular veins.11
Four extensions of the dura mater directly support and separate specific areas of the brain: 1) the falx cerebri, 2) the tentorium cerebelli, 3) the falx cerebelli, and 4) the diaphragma sellae. The falx cerebri divides the right and left hemispheres of the brain vertically through the longitudinal fissures extending from the frontal lobe to the occipital lobe. The tentorium cerebelli forms a tent between the occipital lobes and the cerebellum and separates the cerebral hemispheres from the brainstem and the cerebellum. Structures within the brain that are located above the tentorium are often referred to as supratentorial, whereas those located below the tentorium are referred to as infratentorial and make up the region of the brain called the posterior fossa. The falx cerebelli forms the division between the two lateral lobes of the cerebellum, and the diaphragma sellae forms a roof over the sella turcica, which houses the pituitary gland.1,2
The main blood supply for the dura mater is the middle meningeal artery. This artery lies on the surface of the dura in the epidural space and within grooves formed on the inside of the parietal bone. Traumatic disruption of the parietal bone may result in tearing of the middle meningeal artery and development of an epidural hematoma.12 A potential space exists between the dura mater and the arachnoid mater. This area contains a large number of unsupported small veins that may become disrupted and torn when traumatic head injury occurs, leading to development of a subdural hematoma.12
Arachnoid Mater.
The arachnoid membrane is a delicate, fragile membrane that loosely surrounds the brain. Fine threads of elastic tissue called trabeculae connect the arachnoid to the pia mater, creating a spongy, weblike structure called the subarachnoid space.11 Cerebrospinal fluid (CSF) circulates freely in the subarachnoid space, which also contains the origins of the brain’s large arteries where they enter the skull and differentiate into anterior and posterior circulatory branches.1,2,13 Rupture of an artery within the subarachnoid space allows for blood to mix with CSF, producing a subarachnoid hemorrhage.12
At the base of the brain, widened areas of subarachnoid space form cisterns, or spaces, that are filled with CSF. The largest of these cisterns, the cisterna magna, lies between the medulla and the cerebellum, and it communicates with the fourth ventricle.1,2
Tufts of arachnoid membrane, called arachnoid villi, or granulations, project into the superior sagittal and transverse venous sinuses. Absorption of CSF by arachnoid villi allows its removal by the venous drainage system.1,2,4 The delicate structure of the arachnoid villi places them at risk for obstruction by blood in subarachnoid hemorrhage, resulting in obstructive hydrocephalus.14
Pia Mater.
The pia mater adheres directly to brain tissue. Rich in small blood vessels that supply a large volume of arterial blood to the CNS, this membrane closely follows all folds and convolutions of the brain’s surface. Tufts or folds of the pia mater in the lateral, third, and fourth ventricles form a portion of the choroid plexus that is responsible for the production of CSF.1,3
Ventricular System
The ventricular system consists of four CSF-filled canals lined with ependymal cells, a type of neuroglial cell (Fig. 22-7). This system is made up of two large lateral ventricles that each lie within a hemisphere of the cerebral cortex. Extending from the frontal lobes to the occipital lobe, the lateral ventricles consist of a body, an atrium, and frontal, temporal, and occipital horns.1–3,13 When cannulation of the ventricular system is required for intracranial pressure monitoring, CSF drainage, or placement of a CSF shunt, the frontal horn of the lateral ventricle on the nondominant side of the brain is most often selected.15,16
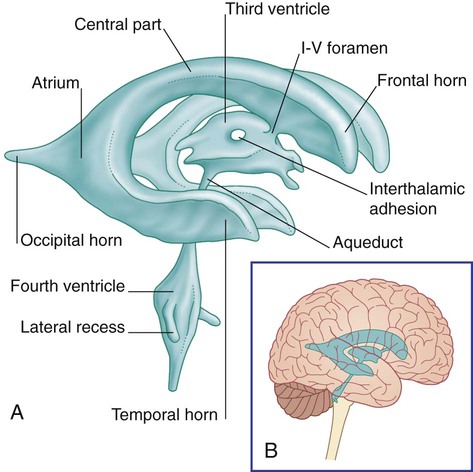
The foramen of Monro connects the two lateral ventricles with a central cavity, the third ventricle. Located directly above the midbrain, the walls of the third ventricle are formed by the thalami. The cerebral aqueduct (aqueduct of Sylvius) is the canal between the third and fourth ventricle, which lies between the brainstem and the cerebellum. At the base of the fourth ventricle, two openings—the foramen of Luschka and the foramen of Magendie—open into the subarachnoid space.1,2 Blockage of CSF flow occurring within the ventricular system obstructs the normal circulation of CSF, causing dilation of the ventricles, a condition called obstructive hydrocephalus.14
Cerebrospinal Fluid
CSF fills the ventricular system and surrounds the brain and spinal cord in the subarachnoid space. Protection of the CNS is further provided by CSF, which acts as a shock absorber when energy is displaced in traumatic injury. CSF is normally clear, colorless, and odorless. It is secreted by the choroid plexuses of the ventricular system, although small amounts are also synthesized by capillaries of the pia mater. Believed to be a filtrate of blood, CSF contains some unique properties that make its synthesis a mystery (Table 22-2).1,2,13
TABLE 22-2
NORMAL VALUES FOR CEREBROSPINAL FLUID
PROPERTY | VALUES |
pH | 7.35-7.45 |
Specific gravity | 1.007 |
Appearance | Clear and colorless |
Cells | 0 white blood cells (WBCs)/mm3; 0 red blood cells (RBCs)/mm3; 0-10 lymphocytes/mm3 |
Glucose | 50-75 mg/dL (two thirds of blood sugar value) |
Protein | 5-25 mg/dL |
Volume | 135-150 mL |
Pressure | 70-200 mm H2O (lumbar puncture); 3-15 mm Hg (ventricular) |
The production of CSF occurs at a rate of approximately 20 milliliters per hour (mL/hr), or 500 mL/day. With a circulating volume of 135 to 150 mL, CSF must be regularly resorbed to prevent development of hydrocephalus. Resorption through intact arachnoid villi is favored by increased hydrostatic pressure mechanics that maintain CSF volume within normal limits. The flow of CSF begins in the lateral ventricles, moves through the foramina of Monro into the third ventricle, moves through the cerebral aqueduct into the fourth ventricle, and moves out the foramen of Magendie and the foramina of Luschka into the subarachnoid space of the brain and spinal cord (Fig. 22-8).1,2,3,13
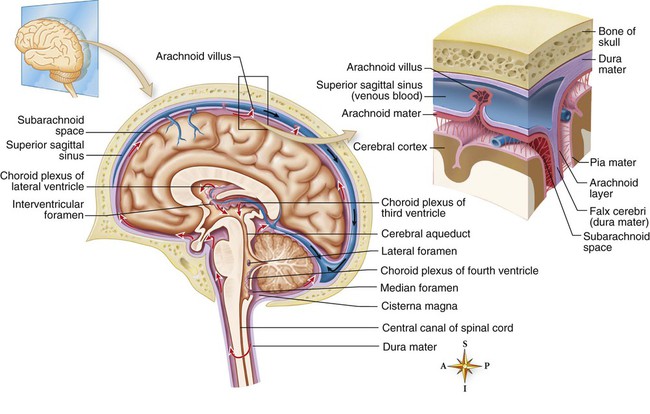
Blood–Brain Barrier
The blood–brain barrier is a physiologic mechanism that helps maintain the delicate metabolic balance in the CNS. The blood–brain barrier regulates the transport of nutrients, ions, water, and waste products through selective permeability.1,2,17
Stabilization of the physical and chemical environment surrounding the neurons of the CNS is the task of the blood–brain barrier. Many substances such as metabolites or toxic compounds cannot cross the blood–brain barrier. Other substances such as antibiotics cross slowly, resulting in lower concentrations of them in the brain than in other areas of the body.17
The blood–brain barrier operates on the concept of tight junctions between adjacent cells, and it consists of three separate barriers.1,3 The vascular endothelial barrier is formed by tight junctions between the endothelial cells of cerebral blood vessels. The blood–CSF barrier consists of tight junctions between the epithelial cells of the choroid plexus. The arachnoid barrier is created by tight junctions between the cells that form the outermost layer of the arachnoid mater. The selective permeability of the blood–brain barrier keeps out toxic or harmful compounds and protects neuronal function.18
Passage of substances across the blood–brain barrier is a function of particle size, lipid solubility, and protein-binding potential. Most medications or compounds that are lipid soluble and stable at body pH rapidly cross the blood–brain barrier. The blood–brain barrier is also highly permeable to water, oxygen, carbon dioxide, and glucose.18
The blood–brain barrier exists only in certain areas of the CNS. The areas in which it does not exist—the pineal region, the basal hypothalamus, and the floor of the fourth ventricle—require contact with plasma to sense changes in concentration of glucose and carbon dioxide and the changes in serum osmolality.1 Initiation of feedback mechanisms by the hypothalamus in response to these changes regulates the internal environment of the remainder of the body.4
Of clinical significance, disruption or alteration of blood–brain barrier permeability occurs with injury to brain tissue from trauma, toxic insults, and ischemic injury. Brain irradiation also may alter the permeability of the blood–brain barrier, although intravenously administered chemotherapeutic agents have been shown to have little effect on blood–brain barrier permeability.19
Cerebrum
The cerebrum is the largest portion of the brain, comprising 80% of its weight. It is composed of two cerebral hemispheres (right and left), separated by the longitudinal fissure and connected at the base by the corpus callosum (Fig. 22-9B).1,2,20
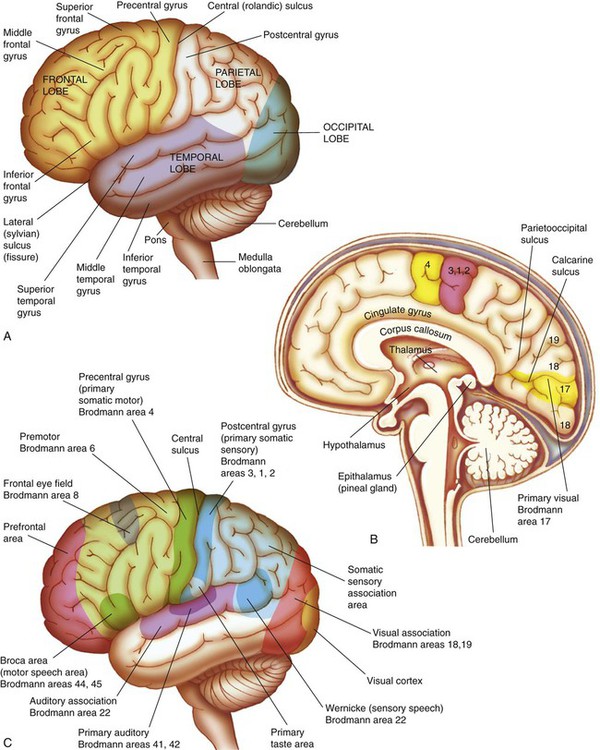
The outermost aspect of the cerebrum is called the cerebral cortex and is made up of gray matter, consisting of neuronal cell bodies. Directly below the cerebral cortex lies white matter, consisting of myelinated axons, which communicate impulses from the cerebral cortex to other areas of the brain. White matter tracts consist of three types of fibers: 1) commissural (transverse), 2) projection, and 3) association.1,2,20 Commissural fibers are tracts that communicate between the two cerebral hemispheres, and the corpus callosum is the largest commissural tract. Projection fibers communicate between the cerebral cortex and the lower regions of the brain and the spinal cord. Association fibers communicate between various regions of the same hemisphere.1,2,20
The cerebral hemispheres are divided into the frontal, parietal, temporal, and occipital lobes (see Fig. 22-9A). The rhinencephalon is often labeled the fifth lobe of the cerebral cortex. Lying deep inside the cerebrum and anatomically associated with the temporal lobe, the rhinencephalon is sometimes referred to as the limbic lobe.1,2,20
The primary functions of the cerebral cortex include sensory, motor, and intellectual (cognitive) functions, making this area of the brain vital to normal human functioning and providing capabilities that make humans unique as a species.3,4,20 Brodmann’s classification of cerebral cortical cytoarchitecture identifies more than 100 unique areas and provides a useful way to localize specific cortical functions within the brain (see Fig. 22-9C).3 This section covers the areas within Brodmann’s classification that are commonly assessed in relation to the development of specific neurologic pathology.
Frontal Lobe
The frontal lobe lies underneath the frontal bone of the skull and is separated posteriorly from the parietal lobe by the central sulcus (fissure of Rolando) and inferiorly from the temporal lobe by the lateral fissure (Sylvian fissure) (see Fig. 22-9A). The major functions of the frontal lobe are voluntary motor function, cognitive function (orientation, memory, insight, judgment, arithmetic, and abstraction), and expressive language (verbal and written).1,2,20
The prefrontal areas (see Fig. 22-9C), located just behind the frontal bone’s distribution over the forehead, are concerned with cognition.3,4 These areas work in concert with other areas of the brain to intellectually appraise and respond to environmental information or stimuli. They augment the intellect with socially trained emotional responses learned over the course of childhood and young adulthood, and they participate in triggering autonomic nervous system responses such as tachycardia in relation to situational needs.3,4 The location of the prefrontal cortex makes it vulnerable to traumatic injury, often resulting in profound changes in cognitive capacity and social responses to environmental stimuli after brain injury.21
The motor strip of the frontal cortex is represented by Brodmann area 4 (see Fig. 22-9C) and consists of cell bodies for neurons associated with voluntary (pyramidal) motor functions. Because most voluntary motor tracts cross over to the opposite side as they descend through the brainstem, the right motor strip represents voluntary motor function for the left side of the body, and vice versa.3,4 The motor homunculus (Fig. 22-10B) is a graphic representation of the distribution of voluntary motor function throughout area 4. Appearing as an upside-down man, the foot of the homunculus is illustrated on the superior medial aspects of the frontal lobes, with the knees, hips, trunk, and shoulders extending along the lateral surfaces and with the hands, thumb, head, face, and tongue represented in a lateral inferior distribution extending to the Sylvian fissure. The homunculus demonstrates larger body part size to denote areas with greater representation because of the amount of dexterity associated with the part’s function. The large surface area of the trunk occupies a relatively small part of the motor strip, whereas smaller body areas such as the thumb or tongue, which involve a great deal of dexterity and fine motor movement, occupy a larger area of the motor strip.3 Damage to the motor strip results in compromise of motor function on the opposite side of the body.22
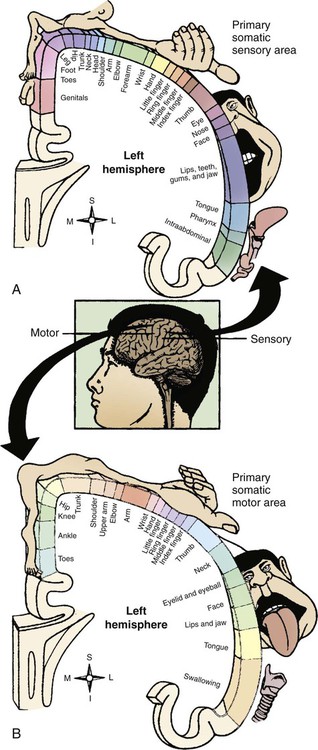
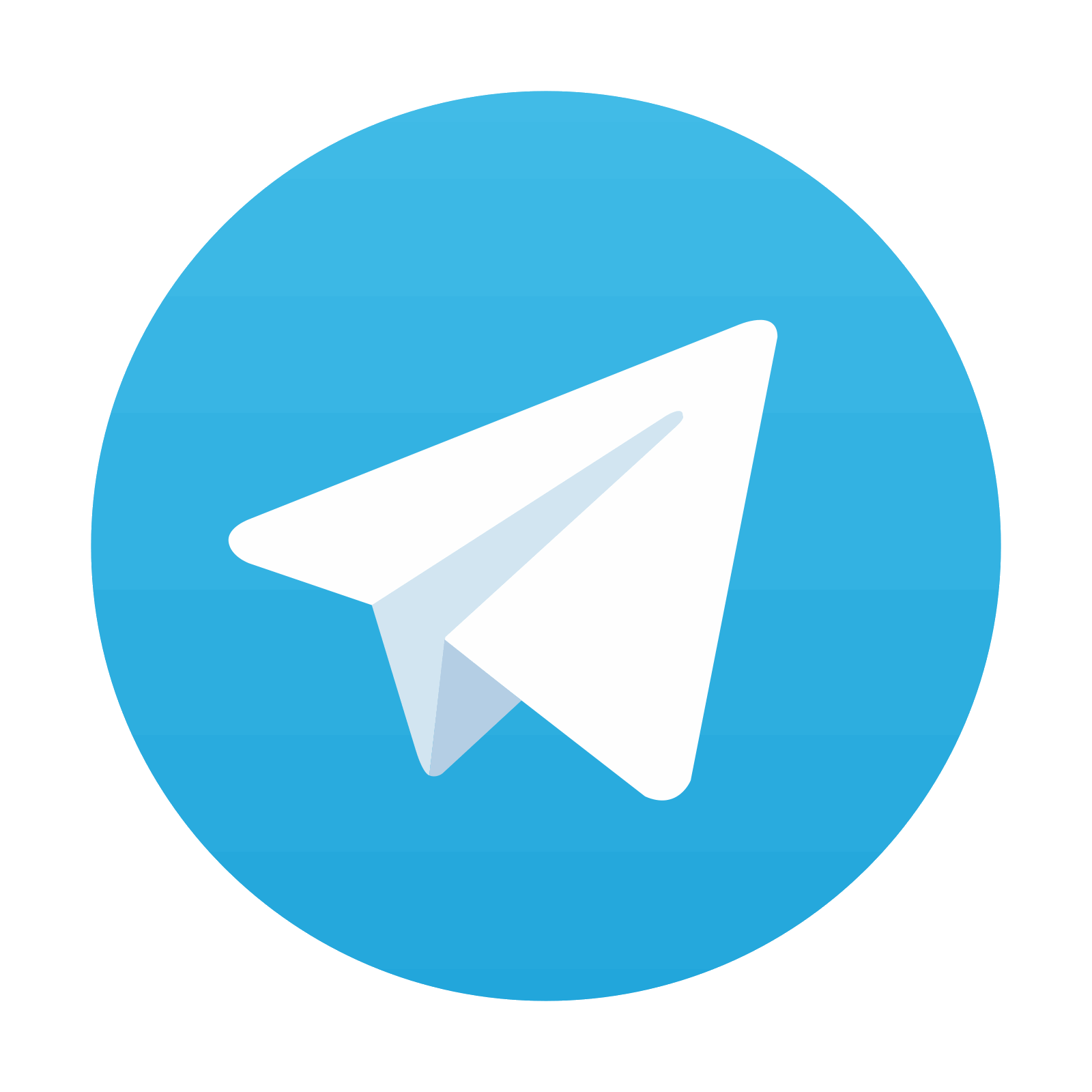
Stay updated, free articles. Join our Telegram channel
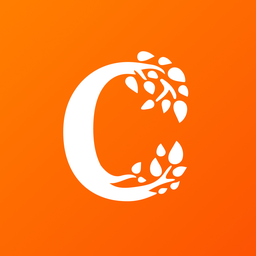
Full access? Get Clinical Tree
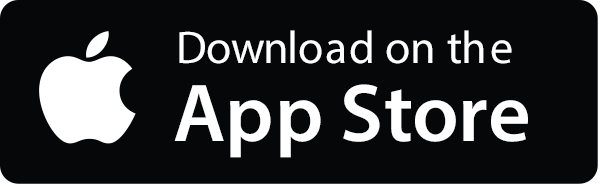
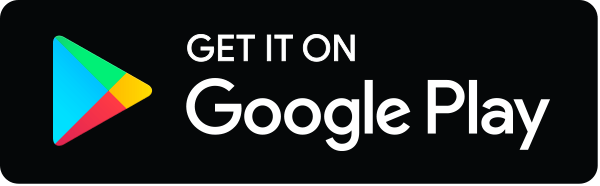