Communicating
Congenital
Achondroplasia
Associated with craniofacial syndromes
Acquired
Posthemorrhagic: intraventricular or subarachnoid
Choroid plexus papilloma or choroid plexus carcinoma
Venous obstruction as in superior vena cava syndrome
Postinfectious
Noncommunicating
Congenital
Aqueductal stenosis
Congenital lesions (vein of Galen malformation, congenital tumors)
Arachnoid cyst
Chiari malformations either with or without myelomeningocele
X-linked hydrocephalus
Dandy-Walker malformation
Acquired
Aqueductal gliosis (posthemorrhagic or postinfectious)
Space-occupying lesions such as tumors or cysts
Head injuries
2.5.1 Communicating Hydrocephalus
Communicating hydrocephalus is a condition that results when the arachnoid villi are unable to adequately reabsorb cerebral spinal fluid. Intraventricular or subarachnoid hemorrhage may cause the arachnoid villi to become unable to function adequately, either temporarily or permanently. This is a consequence of the effect of the end products of red blood cell breakdown on the arachnoid villi. Infectious processes such as meningitis may also render the arachnoid villi to be nonfunctional (due to, e.g., toxins or scarring). Communicating hydrocephalus may also be due to the overproduction of CSF. This is rare and is usually associated with a choroid plexus papilloma or a choroid plexus carcinoma.
2.5.2 Noncommunicating Hydrocephalus
Noncommunicating hydrocephalus is a condition that results when the ventricular system does not communicate with the arachnoid villi due to some obstruction in the normal pathways of CSF flow. Consequently, CSF is produced in the ventricular system but cannot flow to the arachnoid villa to be reabsorbed. Such obstruction can occur when pathways are blocked by a tumor, congenital abnormalities of the brain, cysts, inflammation from infection, or any other condition that interferes with the patency of these pathways. Some consider the failure of the arachnoid villi to reabsorb CSF to be an obstruction at the level of the arachnoid villi.
2.5.3 Congenital Hydrocephalus
Congenital hydrocephalus is caused by any condition that existed before birth. The hydrocephalus may or may not be present at birth. Examples include aqueductal stenosis, Dandy-Walker malformation, and X-linked hydrocephalus. Congenital hydrocephalus is also associated with myelomeningocele, Chiari malformations, encephalocele, and prenatal infections such as cytomegalo inclusion virus (CMV) or rubella.
2.5.4 Acquired Hydrocephalus
Acquired hydrocephalus is hydrocephalus resulting from a condition that did not previously exist in the patient. The condition either obstructs normal spinal fluid flow, causes overproduction of CSF, or prevents reabsorption of CSF. Examples include tumors that obstruct CSF flow and other space-occupying lesions that were not congenital. Infection in the brain may also occlude small passageways. Overproduction of spinal fluid may be caused by a choroid plexus tumor. Acquired conditions that interfere with reabsorption of CSF include intraventricular hemorrhage (IVH) and subarachnoid hemorrhage.
2.5.5 Internal Hydrocephalus
Internal hydrocephalus refers to ventricular dilation and the associated pathophysiology. The term hydrocephalus is used most commonly to refer to internal hydrocephalus.
2.5.6 External Hydrocephalus
External hydrocephalus refers to the accumulation of spinal fluid in either the subarachnoid or subdural spaces. CSF collection in the subarachnoid space may be a benign condition in infancy, which is called benign subdural hygromas of infancy or idiopathic external hydrocephalus of infancy. The neuroimaging characteristics of this condition typically show enlarged frontal subarachnoid spaces and moderately enlarged ventricles. Infants present with rapidly increasing head circumference and sometimes developmental delays. Some children may show temporary or permanent psychomotor delays (Zahl et al. 2011). This is usually a self-limiting condition. The child is usually treated conservatively, although some may require a shunt.
CSF mixed with blood in the subdural space may not be benign and usually requires further investigation and treatment, as it may be related to trauma (possibly nonaccidental trauma). If these fluid collections exert pressure on the brain and cause symptoms or cause very accelerated head growth, surgical treatment may be necessary.
2.5.7 Ex Vacuo Hydrocephalus
Ex vacuo hydrocephalus refers to a condition of brain volume loss. The condition may be present at birth. It may be the result of failure of the fetal development of the brain as in schizencephaly (abnormal development of the brain, leading to the characteristic appearance of abnormal clefts in either one or both cerebral hemispheres) or hydranencephaly (abnormal development of the brain, leading to the absence of the cerebral hemispheres of the brain). The brain may also undergo destruction or atrophy from infections, very poor nutrition, or unknown causes. The ventricles become large to “fill the space” where there is an absence of brain tissue and may or may not be under increased pressure. There is technically not an imbalance of CSF production and absorption but rather the actual loss of brain matter.
2.5.8 Idiopathic Normal Pressure Hydrocephalus
Idiopathic normal pressure hydrocephalus is primarily a condition of the elderly. It is a condition that occurs with normal intracranial pressure and ventricular dilation. These patients develop symptoms slowly over time. The classic symptoms include dementia, gait difficulties, and urinary incontinence. A shunt may be helpful if the symptoms improve after a lumbar puncture or lumbar drain.
2.6 Pathophysiology of Hydrocephalus
2.6.1 Overview of CSF Production and Flow Dynamics
Most of the CSF (approximately 60%) is produced in the choroid plexus; the rest is produced in the ependymal of the cerebral ventricles, the aqueduct of Sylvius, and the subarachnoid space. Studies by Milhorat looking at CSF production after choroid plexectomy demonstrated that the total amount of produced CSF was reduced by only one-third, thus suggesting that other sites can produce larger amount of CSF (Milhorat 1982). He proposed that CSF is also produced as the result of cellular metabolism of periventricular cortical gray matter. These other areas account for 20–50% of CSF production. CSF production requires the expenditure of energy (Albright et al. 2007).
2.6.2 CSF Pathways
CSF flows from the ventricles, passes through a series of channels, and exits the ventricular system via the fourth ventricle. There are two lateral forameni on the lateral aspect of the fourth ventricle, named the foramen of Luschka, and medially located opening called the foramen of Magendie. After exiting the fourth ventricle, the CSF flows into the subarachnoid space and up over the convexities of the brain, to be absorbed into the large intracranial sinuses (Albright et al. 2007). Alternative pathways for CSF have been scientifically supported and include lymphatic drainage into the cervical lymphatic chain and paranasal sinuses. After being absorbed, the CSF is returned to the right atrium via the superior vena cava (Albright et al. 2007).
2.6.3 Intracranial Pressure
A study of rabbits by Dr. McComb found that CSF flows passively and absorption of CSF does not require the expenditure of energy (Albright et al. 2007). For each drop of CSF that is produced, the same amount should be absorbed. Several factors affect the flow of CSF, including resistance, which may result from an obstruction or restriction of a pathway. Other considerations include the plasticity of the brain itself, as well as the flexibility of the intracranial venous structures.
Neuroplasticity refers to the ability of the brain to change in structure or function. For example, an increase in the intraventricular volume will enlarge the ventricles, causing distortion of the cerebral cortex. As we age, our brain may become stiffer. Neonatal brains are very elastic. Anoxic injury can change the brain’s ability to maintain its normal stiffness and can also be hydroplastic. The intracranial venous system includes the dural sinuses which are more rigid than the cortical veins. Cortical veins join the dural sinuses at such an angle that a valvular mechanism is created and a pressure gradient is maintained. The jugular veins, returning the blood to the heart, have no valves. When we stand, negative pressure produced in the jugular veins causes them to collapse and assist humans in maintaining normal intracranial pressure. Shunting systems are used when the CSF pathways are somehow obstructed. The valves that are used to regulate the flow attempt to mimic normal flow.
The normal rate of CSF production in infants and children is about 0.33 ml/kg/h. Normal newborns have about 5 ml total volume of CSF. Adults have about 125 ml of total CSF, with about 20 ml located within the ventricles. CSF is produced continually by the choroid plexus, which is located within the ventricles. It is continually reabsorbed by the arachnoid villi (Figs. 2.1 and 2.2).



Fig. 2.1
Illustration of position and configuration of intracranial ventricles

Fig. 2.2
Illustration of cross section of the brain and ventricles shows pathways of CSF flow
The pathophysiology of hydrocephalus is much more complex than the radiographic picture. The computed tomography (CT) or magnetic resonance imaging (MRI) scan may reveal many structural changes including enlarged ventricles, thinning of the cortical mantle, distortion of structures, and possible transependymal flow of CSF. These visible changes may also affect the biochemistry, metabolism, and maturation of the brain. Adequate treatment and resolution of the dilated ventricles does not always reverse the other injuries that have occurred to the brain.
Three factors are critical in determining the severity of injury caused by hydrocephalus: age at onset, underlying cause (etiology), and duration of the hydrocephalus. Age is a salient factor because hydrocephalus may affect the normal maturation processes of the brain in addition to the other expected effects of increased intracranial pressure. Furthermore, the underlying disease process responsible for the hydrocephalus may have its own destructive effects on maturation and brain function. Examples of such diseases are encephalitis, meningitis, tuberous sclerosis, and tumors. Treatment of these diseases may also have destructive effects on the brain and brain maturation. For example, radiation treatment of brain tumors in very young children can interrupt normal maturation permanently, and development does not always proceed normally, even after the resolution of the hydrocephalus. The duration of the hydrocephalus has a critical role in determining the long-term recovery. Long-standing ventricular dilation and increased intracranial pressure tend to lead to poor recovery of function, even after ventricular size normalizes.
2.6.4 Structural Changes
Ventricular dilation seen on the CT or MRI is the hallmark of hydrocephalus. The temporal and frontal horns of the lateral ventricles usually dilate first and are sometimes asymmetrical. This is due to the accumulation of spinal fluid and leads to distortion of the adjacent structures, compression of the nearby white matter, reduction of cerebral cortex, and thinning of the cortical mantle. The ependymal cells lining the ventricles may become damaged and allow transependymal flow of CSF. The septum pellucidum may become damaged, leading to its disappearance and the formation of one large ventricular cavity. In some situations, ventricular size may not change even though pressure is elevated and symptoms are present.
2.6.5 Vascular Changes
The distortion of the brain tissue that occurs with hydrocephalus also affects the arteries, veins, and capillaries. Deep vessels are affected the most as they may be directly compressed from the increased ventricular size. Peripheral vessels are also affected as they try to supply the brain tissue that is suffering from the insult of increased intracranial pressure. Blood flow has been shown to be globally decreased to the brain in acute hydrocephalus (Da Silva et al. 1995). Blood flow is primarily decreased to the periventricular white matter in chronic hydrocephalus (Da Silva et al. 1995). Hypoperfusion may cause damage to neurons and glia and interfere with normal maturation of all brain structures.
2.6.6 Metabolic Changes
The brain of a child consumes about 50% of total body oxygen, and an infant’s brain consumes more than 50%. The adult brain consumes only about 20% (Sokoloff 1989). The brain uses glucose as its primary source of energy with few exceptions. Therefore, any decrease in cerebral blood flow that decreases the amount of oxygen and glucose available can markedly alter metabolism. This impairment of metabolism may lead to damage to the brain. Furthermore, during infancy and childhood, a significant portion of the energy used by the brain is used for maturational activities such as myelination, neuronal maturation, and protein production. Normal maturation may be disturbed and possibly permanently altered, due to these metabolic alterations.
2.6.7 Cerebral Spinal Fluid Changes
Abnormal amounts of spinal fluid in the brain may lead to changes in the CSF itself. Metabolites may accumulate in the CSF during hydrocephalus. Protein levels in the CSF may be altered by the underlying cause of the hydrocephalus. For example, after an IVH, protein levels may be very elevated. If the hydrocephalus damages the ependymal cells lining the ventricles, the CSF may flow out of the ventricles into the periventricular white matter. CSF production may or may not decrease as intracranial pressure increases. As intracranial pressure increases, reabsorption of CSF may increase assuming that the arachnoid villi are functional.
2.6.8 Brain Tissue Changes
The white matter surrounding the enlarged ventricles is called the periventricular white matter. As the ventricles dilate, the white matter may become compressed, saturated with CSF, and possibly damaged. Periventricular leukomalacia may result from ischemia to affected white matter. The corpus callosum may also become thinned.
The myelination process may also be delayed in children with hydrocephalus. Myelination occurs in a stepwise fashion during development. If one step is interrupted, it cannot occur at a later time, and this prevents subsequent steps in the overall process.
The cerebral cortex is also markedly affected by hydrocephalus. The cortex is thinned as it is pushed out by the ventricles and restricted by the skull. Histological changes within the cortex are usually subtle, but damage to cells occurs and results in a change in function.
The goal of treatment of hydrocephalus is to prevent further damage and to restore function. Treatment usually reverses symptoms of acute hydrocephalus. However, timing is critical, and treatment should occur before vascular, metabolic, and other changes described interfere with normal maturation and brain function. Without prompt treatment, acute hydrocephalus and increased intracranial pressure can lead to brain stem herniation and death.
2.7 Etiologies of Hydrocephalus
Hydrocephalus is primarily a condition of obstructed CSF circulation or absorption. In infants and children, it may be congenital or associated with other congenital abnormalities. It may also be associated with central nervous system (CNS) infection, hemorrhage, tumors, or cysts.
2.7.1 Aqueductal Stenosis
In 1900, Bourneville and Noir noted an association between hydrocephalus and stenosis of the aqueduct of Sylvius (Cinalli et al. 2004). The aqueduct of Sylvius is a narrow passageway connecting the third and fourth ventricles. The most common cause of hydrocephalus in children is aqueductal stenosis, and it accounts for 70% of cases (Greenberg 2010). Hydrocephalus due to aqueductal stenosis is characterized by enlargement of the lateral and third ventricles with a normal fourth ventricle. This constriction of the aqueduct of Sylvius is best seen on MRI scan (sagittal view).
Stenosis of this passageway may be congenital or acquired, although in 50–75% of cases, the cause may be unknown. It may be associated with Chiari I malformation, vein of Galen malformation (VGAM), or Dandy-Walker malformation. Aqueductal stenosis may also be due to an X-linked recessive gene, L1CAM mutation, (Langingham et al. 2009) occurring only in males. This is rare and is associated with characteristic adducted thumbs, spastic paraparesis, and mental retardation. Acquired cases of aqueductal stenosis may be the result of hemorrhage, inflammation from infection, or obstruction from a nearby tumor or cyst.
2.7.2 Myelomeningocele and Chiari II Malformation
Myelomeningocele is a neural tube defect that occurs during embryonic development resulting in failure of the neural tube to close. This malformation involves the entire CNS. At the level of the spinal defect, there is a midline lesion containing meninges, spinal cord, nerves, and CSF. The bony structures of the spine may be abnormal or absent. Associated abnormalities in the brain include Chiari II malformation, hydrocephalus, and possibly other structural abnormalities.
Chiari II malformation occurs in almost all infants born with myelomeningocele.
It is a malformation of the hindbrain, fourth ventricle, and brain stem and includes herniation of these structures into the cervical spinal canal. Herniation of the brain stem and fourth ventricle may result in obstruction of CSF flow. The development of hydrocephalus is related to the Chiari II malformation, aqueductal stenosis, venous hypertension in the posterior fossa, and closure of the myelomeningocele (Sgouros 2004a, b).
Hydrocephalus develops in about 85% of children with myelomeningocele. Approximately 50% have significant hydrocephalus at birth (Wang and Avellino 2005). About 80–90% will eventually require a CSF shunt (Dias 2005) or an endoscopic third ventriculostomy (ETV). Before modern shunting of these infants in the 1960s, only about 20% of non-shunted children lived into adulthood. Today, hydrocephalus can usually be adequately treated. Infants and children who die from this complex condition usually die from the Chiari II malformation and brain stem dysfunction. New research shows that patients who have undergone prenatal closure of the myelomeningocele have lower rates of hydrocephalus, and in some patients, there was no formation of a Chiari II malformation (Adzick et al. 2011).
Myelomeningocele is discussed in detail in Chap. 5.
2.7.3 Chiari I Malformation
Chiari I malformation is one of the four types of Chiari malformations. In Chiari I, the cerebellar tonsils are elongated and herniated into the cervical spinal canal. Chiari I is not associated with myelomeningocele and may be acquired from increased intracranial pressure or occur as an isolated condition.
Hydrocephalus occurs in 10% of children with Chiari I malformation, most likely due to blockage of CSF flow at the craniovertebral junction. A small posterior fossa may also alter CSF flow. Treatment for patients with symptomatic Chiari I malformation is often a posterior fossa decompression. A small percentage of children develop hydrocephalus after the decompression.
Chiari malformations are discussed in detail in Chap. 6.
2.7.4 Dandy-Walker Malformation
Dandy-Walker malformation is a continuum of posterior fossa abnormalities including Dandy-Walker malformation and Dandy-Walker variants. The abnormalities associated with these conditions include cystic dilation of the fourth ventricle, partial or complete absence of the cerebellar vermis, upward displacement of the tentorium, and usually hydrocephalus. Dandy-Walker may be differentiated from a posterior fossa cyst by the atrophy or agenesis of the vermis seen on MRI scan. Dandy-Walker malformation/variant may also be associated with other intracranial abnormalities in 70% of patients. These abnormalities include agenesis of the corpus callosum, aqueductal stenosis, schizencephaly, holoprosencephaly (failure of the prosencephalon, the embryonic forebrain, to sufficiently divide into the double lobes of the cerebral hemispheres, resulting in a single-lobed brain and severe craniofacial defects), neural tube defect, and occipital encephalocele. Dandy-Walker is found in 2–4% of all children with hydrocephalus (Greenberg 2010). Other abnormalities associated with Dandy-Walker malformation/variant include congenital heart defects, renal malformations, polydactyly/syndactyly, cleft palate, perineal malformations, Klippel-Feil malformation, and facial hemangiomas.
Hydrocephalus occurs in 90% of children with Dandy-Walker malformation/variant (Greenberg 2010). Initially, it was believed that associated hydrocephalus was caused by obstruction of the foramens of Luschka and Magendie. Dandy and Blackfan (1914) believed that the foramens failed to develop or were obstructed due to a prenatal inflammatory process. However, in some cases, the forameni are found to be patent. Also, about 80% of infants with Dandy-Walker malformation do not have hydrocephalus at birth (Cinalli et al. 2004). The pathophysiology of hydrocephalus associated with Dandy-Walker is now felt to be multifactorial. Contributing factors include aqueductal stenosis, basal arachnoiditis from an inflammatory process, abnormally developed subarachnoid space, and venous hypertension from direct pressure from the posterior fossa cyst (Cinalli et al. 2004) (Fig. 2.3).


Fig. 2.3
A 2-month-old female with Dandy-Walker malformation. MRI shows a posterior fossa cyst of the fourth ventricle and subsequent development of severe hydrocephalus
2.7.5 Vein of Galen Malformation
A vein of Galen malformation is a rare vascular malformation. It is a venous aneurysm of the vein of Galen fed by numerous aberrant branches of the carotid or vertebrobasilar vessels. In addition, arteriovenous malformations may occur within the feeding vessels.
Infants with a vein of Galen malformation often present at birth with congestive heart failure and hydrocephalus. They may also develop hydrocephalus later. Hydrocephalus may be caused by the venous malformation causing obstruction of the cerebral aqueduct. Elevated intracranial venous pressure may also decrease CSF reabsorption and cause hydrocephalus (Fig. 2.4a, b).


Fig. 2.4
A 4-month-old male with vein of Galen malformation: (a) MRI shows the dilated vein of Galen; (b) cerebral angiogram shows the dilated vein of Galen and the surrounding vasculature
Cerebrovascular diseases are discussed in detail in Chap. 12.
2.7.6 Arachnoid Cysts
An arachnoid cyst is a benign congenital cyst occurring within the brain. The cyst forms during fetal development with the splitting of the arachnoid membrane (Raffel and McComb 1994), creating an intra-arachnoid space and the resultant cyst. Most of these cysts do not change or cause any other problems. Such cysts are often found incidentally when a child has a scan for some other reason (i.e., head injury). If the cyst enlarges, it may compress the surrounding structures and cause symptoms from mass effect. Depending on the location, as the cyst expands, it may compress nearby CSF pathways and cause hydrocephalus. A suprasellar cyst may expand upward pressing on the floor of the third ventricle and obstruct the foramen of Monro or aqueduct of Sylvius. A cyst in the quadrigeminal cistern or supracollicular region may cause obstruction of the aqueduct of Sylvius. A posterior fossa arachnoid cyst can cause obstruction at the level of the fourth ventricle. A posterior fossa cyst can be differentiated from a Dandy-Walker malformation by the presence of the cerebellar vermis and a normal appearing fourth ventricle on an MRI. The etiology of expansion of the cyst is unknown.
Surgical intervention is required if hydrocephalus occurs or there are symptoms of mass effect from the cyst. What appears to be an arachnoid cyst may also be associated with a brain tumor. Therefore patients with a newly found cyst require a full MRI of the brain, possibly with contrast, to rule out a tumor. An arachnoid cyst identified before the age of two typically presents with macrocephaly. These cysts are thought to be affected by CSF dynamics, requiring a greater rate of treatment. In Zada et al.’s (2007) study, after fenestration, 57% of patients still required shunt placement (Zada et al. 2007) (Fig. 2.5).


Fig. 2.5
MRI shows a large posterior fossa cyst which effaces the aqueduct of Sylvius and fourth ventricle causing severe hydrocephalus
2.7.7 Posthemorrhagic Hydrocephalus of Prematurity
The most common cause of hydrocephalus in the premature infant is a germinal matrix hemorrhage. The germinal matrix is a very vascular area in the fetal brain, in the subependymal region located at the level of the foramen of Monro. It is from the very thin-walled germinal matrix vessels that the bleeding is thought to occur in preterm infants. Bleeding can spread, most often to the adjacent ventricles and into the surrounding parenchyma. The germinal matrix gradually involutes after 34 weeks’ gestation and nearly disappears by 40 weeks. A Papile grading system was devised to describe the severity of the bleeding – Grades I–IV (Wang and Avellino 2005) (Table 2.2).
Table 2.2
Grading of intraventricular hemorrhage
Grade | Extent of hemorrhage (IVH) |
---|---|
I | Subependymal germinal matrix hemorrhage |
II | IVH without ventriculomegaly |
III | IVH with ventriculomegaly |
IV | IVH with parenchymal hemorrhage |
Premature infants less than 34 weeks’ gestation with very low birth weight (<1,500 g) are at greatest risk for developing intraventricular hemorrhage (IVH). With current management, 20% of these preterm infants will develop an IVH (Boop 2004). The risk of developing posthemorrhagic hydrocephalus (PHH) is directly related to the extent of the hemorrhage. Hydrocephalus develops in 9% of infants with IVH (Christian et al. 2016). In infants with Grade III and IV IVH, 25% and 28%, respectively, went on to develop hydrocephalus, whereas in patients with Grade I and Grade II, only 1% and 4%, respectively, developed hydrocephalus (Christian et al. 2016). PHH may develop as a result of the accumulation of blood and hemorrhagic debris within the ventricles and subarachnoid spaces (Fig. 2.6). Obstruction of the aqueduct of Sylvius or foramen of Monro may occur. The breakdown of the blood may also render the arachnoid villi unable to reabsorb the CSF. Multiloculated hydrocephalus may occur after IVH due to ventriculitis. Ventricular septations may develop causing isolated compartments of fluid within the ventricles.


Fig. 2.6
A 25-week premature male with an intraventricular hemorrhage and subsequent development of hydrocephalus: (a) CUS shows the right-sided intraventricular hemorrhage; (b) CT also shows parenchymal hemorrhage
2.7.8 Postinfectious Hydrocephalus
Intracranial infection at any age may cause hydrocephalus. Hydrocephalus may follow bacterial, fungal, viral, and parasitic infections of the CNS. In utero, CNS infections may cause intracranial injury leading to obstruction of CSF flow. Toxoplasmosis may cause inflammation and blockage of the CSF pathways and blockage within the subarachnoid spaces (Ciurea et al. 2004). During the neonatal period, gram-negative bacteria are the leading cause of bacterial meningitis (Ciurea et al. 2004). Gram-negative bacteria may also cause ventriculitis (Ciurea et al. 2004) leading to hydrocephalus.
After the neonatal period, gram-positive bacteria are the leading cause of meningitis. Meningitis and ventriculitis may lead to multiloculated hydrocephalus, a condition where noncommunicating pockets of CSF occur within the ventricles. Viral infections, including CMV, parainfluenza, and influenza A, can affect ependymal cells leading to acquired aqueductal stenosis and hydrocephalus (Ciurea et al. 2004). Tuberculosis meningitis may cause obstructive hydrocephalus from mass effect of a tuberculoma or cause a communicating type of hydrocephalus by affecting the basal cisterns. Hydrocephalus may develop in conjunction with the intracranial infection or much later after recovery.
Cysticercosis occurs throughout the world. It is uncommon in the United States but is found throughout Central/South America. Humans can acquire the pork tapeworm, Taenia solium, by eating undercooked pork or by consuming the tapeworm eggs from food contaminated with human feces. The tapeworm larva enters the body and forms cysticerci. Neurocysticercosis results when the cysts enter the brain. The cysts can implant in the parenchyma, ventricles, subarachnoid space, or cisterns. Hydrocephalus can occur when cysts are in the ventricles, subarachnoid space, and cisterns or cause arachnoiditis. In areas such as southern California, Arizona, and New Mexico, neurocysticercosis must be considered as an etiology of hydrocephalus.
2.7.9 CNS Tumors
Hydrocephalus can be a complicating factor of pediatric brain tumors. It may be present at the time of diagnosis of the tumor, may occur during or after tumor treatment, or may develop if the tumor reoccurs. Most of the time, hydrocephalus associated with tumors is due to the obstruction of CSF pathways.
About 60% of brain tumors in children are located infratentorially or in the posterior fossa, occurring in the cerebellum, fourth ventricle, or brain stem. The most common tumors of this region include medulloblastoma, astrocytoma, and ependymoma. Hydrocephalus is common with tumors in this area. It results from obstruction of CSF flow, particularly if the tumor is in the fourth ventricle or exerting pressure on the fourth ventricle. A tectal plate tumor is an indolent tumor of the midbrain and results in hydrocephalus. In all of these tumors, hydrocephalus is often a major contributor to symptoms at the time of diagnosis. If the hydrocephalus is severe, urgent treatment is needed to relieve increased intracranial pressure. Hydrocephalus may also occur from blood and debris in the CSF after tumor resection. Approximately 25–50% of children will require placement of a permanent shunt (Wang and Avellino 2005) or endoscopic third ventriculostomy after the tumor resection. Certain factors are associated with the need for permanent CSF diversion including age less than 10 years, midline tumors, incomplete tumor resection, CSF infection, and persistent pseudomeningocele (Sainte 2004).
About 40% of pediatric brain tumors occur in the supratentorial area. The most common site is the suprasellar region, followed by the cerebral hemispheres, thalamus and basal ganglia, pineal region, intraventricular spaces, and meninges. Hydrocephalus is associated with some of these tumors and is usually due to obstruction of CSF flow at the aqueduct of Sylvius. Tumors in the suprasellar region most commonly associated with hydrocephalus are craniopharyngioma and optic pathway glioma. Craniopharyngiomas can also form cysts that exert mass effect that causes symptoms and/or hydrocephalus. Pineal region tumors are commonly associated with hydrocephalus. Tumors that grow within the ventricles may cause hydrocephalus as a result of overproduction of CSF. There are two types of choroid plexus tumors: choroid plexus papilloma and choroid plexus carcinoma. They arise from the choroid plexus, located within the lateral, third, and fourth ventricles. Occasionally, germ cell tumors and pituitary adenomas may cause hydrocephalus. Hydrocephalus may also occur in patients with neurofibromatosis or tuberous sclerosis secondary to obstruction of CSF flow.
Spinal cord tumors are rare in children. They may be associated with hydrocephalus due to arachnoiditis and elevated protein in the CSF (Fig. 2.7).


Fig. 2.7
(a, b) An 8-year-old female with a posterior fossa brain tumor and hydrocephalus
Brain and spinal cord tumors are discussed in detail in Chap. 7.
2.7.10 Head Trauma
Hydrocephalus may occur after head injury if there is intracranial blood. This is particularly true if there is subarachnoid hemorrhage or IVH. The breakdown of the blood may alter the ability of the arachnoid villi to absorb CSF. Debris and blood may also obstruct normal CSF pathways and cause obstructive hydrocephalus.
2.8 Signs and Symptoms of Hydrocephalus
The signs and symptoms of hydrocephalus in infants and children vary depending on their age, the degree of hydrocephalus at presentation, the primary etiology, and the time over which the hydrocephalus develops. Because of the plasticity of the infant brain and the ability of the cranium to expand, ventriculomegaly can progress without obvious signs of increased intracranial pressure. In premature infants, in which hydrocephalus is caused predominately by IVH, there is a general correlation between the severity of hemorrhage and the degree of hydrocephalus (Table 2.3). Infants with PHH may have minimal symptoms or may exhibit increasing spells of apnea and bradycardia. They may also have hypotonia, sunsetting eyes, ophthalmoplegia, and seizures. As the ventriculomegaly progresses, the fontanel will bulge become tense and nonpulsatile, and the cranial sutures become splayed. In a healthy premature infant, the head circumference generally increases about 1 cm a week. In premature infants with progressive ventriculomegaly, the head circumference may increase more rapidly than normal (when charted on the head growth chart) but may not accurately reflect the rate of increase in ventricular size.
Table 2.3
Signs and symptoms of hydrocephalus in children
Premature infants | Full-term infants | Toddlers and older |
---|---|---|
Rapid head growth | Macrocephaly | Headache |
Tense fontanelle | Tense fontanelle | Nausea |
Apnea | Rapid head growth | Vomiting |
Bradycardia | Splayed cranial sutures | Irritability |
Splayed cranial sutures | Decreased feeding | Lethargy |
Sunsetting eyes | Vomiting | Delayed development |
Vomiting | Distended scalp veins | Decreased school performance |
Hypotonia | Increased drowsiness | Behavioral disturbance |
Acidosis | Poor head control | Papilledema |
Seizures | Parinaud’s sign | Parinaud’s sign |
Sunsetting eyes | Sunsetting eyes | |
Frontal bossing | Bradycardia | |
Hypertension | ||
Irregular breathing patterns |
In full-term infants, signs often include macrocephaly and progressively increasing occipital frontal head circumference, crossing percentile curves. Normal head circumference for a full-term infant is 33–36 cm at birth. A normal head circumference increases by approximately 2 cm/month during the first 3 months, by 1.5 cm/month during the fourth and fifth months, and by about 0.5 cm/month from months 6 to 12 (Fig. 2.8).


Fig. 2.8
Growth charts show the head circumference rapidly crossing percentile curves: (a) premature male infant growth chart and (b) full-term male growth chart
Other common signs in full-term infants include a bulging, tense anterior fontanel; splayed cranial sutures; irritability; poor feeding; episodes of spitting up or vomiting; increased sleeping; distended scalp veins; and, if the head is large relative to size, poor head control. Visual changes may also be noted and include paralysis of upward gaze (Parinaud’s sign) and sunsetting eyes.
Children older than 2 or 3 years may have a more acute presentation of symptoms since the cranial fontanels and sutures are closed, and the skull is no longer able to compensate for the increasing ventricular size. The predominant symptom is usually a persistent headache that typically occurs upon wakening and is often associated with nausea, vomiting, and lethargy. The child is often irritable. A child who has a gradual onset of hydrocephalus may have more subtle signs, such as delayed development in both motor and cognitive functions. Older children often present with decreased school performance and behavioral disturbance. Other less common signs may include papilledema and visual complaints. If hydrocephalus is severe, Cushing’s triad of bradycardia, systemic hypertension, and irregular breathing patterns may occur. This triad denotes a severe case of increased intracranial pressure and requires emergent treatment.
2.9 Diagnosis of Hydrocephalus by Imaging Studies
The three major techniques used for diagnosis and evaluation of hydrocephalus are ultrasonography (US), CT, and MRI.
2.9.1 Ultrasonography
Prenatal US can be highly reliable and accurate in diagnosing hydrocephalus. Hydrocephalus can be detected in a fetus as early as the later part of the first trimester of pregnancy, although abnormal dilation of the fetus’ ventricles are more clearly detectable after 20–24 weeks’ gestation (University of California, San Francisco 2000). Although prenatal US can detect an abnormal CSF collection, it may not show the precise site or cause of obstruction. Amniocentesis can often detect the presence of open neural tube defects, such as myelomeningocele, chromosome abnormalities, and in utero infections, and may also help indicate the overall health of the fetus. In general, the first trimester development of significant hydrocephalus can be a poor prognostic sign for infant mortality and developmental progress. In some cases, mild ventricular dilation identified by US will resolve by the third trimester (University of California, San Francisco 2000).
Cranial US (CUS) is useful in infants and young children while the anterior fontanel is still open, usually under the age of 18 months (Fig. 2.9). Through the open fontanel, CUS can demonstrate lateral ventricular morphology and intraventricular clots. It is less accurate in its ability to look at the third and fourth ventricles and subarachnoid spaces. For this reason, the precise diagnosis and cause of hydrocephalus are rarely made by CUS alone. It is particularly useful, however, for follow-up screening of infants with untreated and treated hydrocephalus. The equipment is portable, involves no radiation, does not require sedation, and is considerably less expensive than CT/MRI.


Fig. 2.9
(a) Normal CUS in a 1-month-old female and (b) hydrocephalus in a 3-week-old male
2.9.2 Computed Tomography (CT)
Since the advent of CT scanning in 1976, it is a common radiologic technique for the diagnosis and follow-up of hydrocephalus. CT images can accurately demonstrate the ventricular size and shape the presence of blood and calcifications, cysts, and shunt hardware. With hydrocephalus, an enlarged ventricular system is usually seen and is typically first seen in the lateral ventricles (Fig. 2.10). CT images can also accurately reflect signs of increased intracranial pressure, such as compressed cerebral sulci, absent subarachnoid spaces over the convexity, and transependymal reabsorption of CSF into the white matter. When contrast enhancement is used, tumors, abscesses, and some vascular malformations can be visualized. It is a diagnostic screening tool, taking only a few minutes, and few children need to be sedated for the procedure. CT does expose the patient to radiation, and little is known about the long-term effects of multiple scans. Many providers have concern about the effects of repeated CT scan on the growing brain. Alternatives include low-dose CT or HASTE (limited T2) MR scans. These are acceptable methods for following ventricular size. The Alliance for Radiation Safety has created the “Image Gently” campaign (www.imagegently.com) to assist providers in obtaining the images they need while decreasing the radiation exposure to the patient.


Fig. 2.10
(a) Normal CT in a 9-year-old male and (b) hydrocephalus in a 2-week-old male
2.9.3 Magnetic Resonance Imaging (MRI)
Commercially available MRI was introduced in 1986 and is the examination of choice for revealing the underlying cause of hydrocephalus. It allows anatomical visualization in the axial, coronal, and sagittal planes, providing detailed information regarding the anatomy and the position and extent of lesions. Subtle findings, such as white matter pathology, dysmorphic anatomy, and characteristics of lesions, can be readily demonstrated. In addition, the aqueduct of Sylvius can be visualized, as well as membranes and loculated ventricular systems. With the addition of gadolinium (an intravenous contrast medium), some neoplasms, infectious and vascular lesions, can be better visualized. CSF flow dynamics can be visualized through the use of phase-contrast cine MRI. This sequence takes only a few extra seconds and allows for real-time flow measurements that are demonstrated on the sagittal plane of the MRI. Furthermore, constructive interference in the steady state (CISS) sequence MRI may be used. This sequence provides great detail of the ventricular system and basal cisterns and may show membranes not otherwise seen on conventional MRI. Both phase-contrast cine MRI and CISS sequence MRI can be very helpful in determining the underlying cause of hydrocephalus. They can also provide valuable preoperative information related to the potential success of endoscopic third ventriculostomy, as well as postoperative information by being able to visualize the CSF flow pattern. MRI takes approximately 45 min or longer, and therefore, young children need to be sedated or anesthetized for the exam. Typically developing children over the age of 5 or 6 can often do the exam without sedation.
The half-Fourier single-shot turbo spin-echo (HASTE) MRI is a limited T2 image that provides good visualization of ventricular anatomy. It is an alternative to CT, does not expose the child to radiation, and requires no sedation as it is a short study lasting less than 20 s to 3 min (O’Neill et al. 2013).
Aqueductal stenosis and hydrocephalus are shown in Figs. 2.11, 2.12, and 2.13, along with cine and CISS MRI scans.




Fig. 2.11
A 13-year-old male with aqueductal stenosis and hydrocephalus: (a) Sagittal T1 MRI shows enlarged lateral ventricles (CSF appears black); (b) coronal T2 MRI shows enlarged lateral and third ventricles (CSF appears white)

Fig. 2.12
A 13-year-old male with hydrocephalus secondary to aqueductal stenosis, status post endoscopic third ventriculostomy (ETV). CSF cine flow study demonstrates CSF flow across the fenestration in the anterior third ventricle: (a) phase-contrast magnitude cine MRI and (b) phase-contrast directional cine MRI

Fig. 2.13
A 13-year-old male with hydrocephalus secondary to aqueductal stenosis. Preoperative CISS (constructive interference in the steady state) MRI demonstrates the floor of the third ventricle and the position of the basilar artery
2.10 Treatment of Hydrocephalus
2.10.1 Medical Therapy
There is currently no medical therapy that definitively treats hydrocephalus effectively. Occasionally, in borderline cases of progressive hydrocephalus and in PHH, diuretics may be useful as a temporizing measure to try to avoid the need for a permanent shunt. Acetazolamide, a carbonic anhydrase inhibitor, has been shown to decrease CSF production. The dose may be as high as 100 mg/kg/day, and in order for it to be effective, more than 99% of carbonic anhydrase must be blocked before CSF production decreases significantly. Furosemide, 1 mg/kg/day, has also been used. The mechanism of action is unknown, but it is thought to decrease brain extracellular fluid. Although these have been used historically as temporizing measures, comprehensive analysis of data from clinical trials on diuretic therapy for PHH by the Cochrane Collaboration concluded that acetazolamide and furosemide were neither effective nor safe for the treatment of PHH (Whitelaw et al. 2001).
Serial lumbar or ventricular punctures to evacuate CSF are also used as a temporizing measure. The efficacy of these punctures is controversial, but some centers routinely use them in infants until they are stable enough to tolerate a surgical intervention. The goals are to decrease the ICP and help clear the CSF of toxic chemicals produced by the breakdown of blood. If the infant continues to have inadequate CSF reabsorption, a more permanent shunt may be implanted.
2.10.2 Surgical Intervention
2.10.2.1 Shunts
CSF shunting is the most common standard treatment in the long-term management of hydrocephalus. It involves the placement of a ventricular catheter to divert CSF to another body cavity, where it can be absorbed. There are many different shunting devices with different components, all having similar features. The three main components of a shunt are a proximal (ventricular) catheter, a valve, and a distal catheter (Fig. 2.14). The ventricular catheter is a Silastic tube that is placed either through a frontal or parieto-occipital approach, usually in the right nondominant cerebral hemisphere, as shown in Fig. 2.15. via a burr hole.



Fig. 2.14
Shunt components (Courtesy of Medtronic Neurologic Technologies)

Fig. 2.15
Illustration demonstrates that proximal catheter placement is generally through a frontal or occipital approach
There are many different valves made by many different manufacturers. They all regulate the flow of CSF by means of a one-way valve. The valves most commonly used in the pediatric setting today are differential pressure valves, flow-regulating valves, and siphon-resisting valves. The pressure at which the valves open is termed the opening pressure. Typically there are low-, medium-, and high-pressure valves in each category, referring to opening pressures of approximately 5, 10, and 15 cm H2O, respectively. Most valves are differential pressure valves and are designed to open and allow drainage of CSF as the intraventricular pressure rises above the valve’s opening pressure. Once the pressure falls below the closing pressure, the valve closes and the flow of CSF ceases. Flow-regulating valves attempt to keep the CSF flow constant despite changing pressure differentials and patient position. Siphon-resisting valves are used to avoid siphoning of CSF and the complication of over-drainage. Siphoning is a phenomenon that occurs in some patients in whom there is gravity-enhanced flow of CSF when the patient is in an upright position. The choice of which valve to use is based on the personal preference of the neurosurgeon and is usually based on training and personal experience. No data exists to support a recommendation of one particular shunt design or valve over another. Fixed pressure valves are shown in Fig. 2.16.


Fig. 2.16
(a) Fixed pressure valves and (b) delta valves with siphon control (Courtesy of Medtronic Neurologic Technologies)
A recent advance in shunt valve technology has been the introduction of programmable valves (Figs. 2.17, 2.18, 2.19, 2.20, and 2.21). Programmable valves allow the opening pressure of the valve to be adjusted externally with the use of a special magnetic device. This avoids the need for an operative procedure should the patient need a valve with a different pressure. This type of valve tends to be well suited for the management of difficult cases of over-drainage or underdrainage or in children whose pressure needs are expected to change over time. It is not clear that the benefits outweigh the increased cost in all patients. Since the programmable valve contains a magnet, most valves need to be reprogrammed immediately after all MRIs. Several programmable valves that are not altered by a magnetic field are also available. In these valves, the setting is locked and can only be changed with the manufacturer’s specific magnetic programmer. Special precautions should be taken when the patients are around strong magnetic sources; patients and families should be directed to the manufacturer’s recommendations on magnetic considerations.






Fig. 2.17
(a) Codman Hakim programmable valves, (b) strata programmable valves, and (c) Certas valves (Courtesy of Medtronic Neurologic Technologies/Codman)

Fig. 2.18
Codman Hakim valve programmer

Fig. 2.19
Illustration demonstrates programming a Codman Hakim programmable valve

Fig. 2.20
Strata valve programmer (Courtesy of Medtronic Neurologic Technologies)

Fig. 2.21
Certas valve programmer (Courtesy of Codman)
Distal catheters are also made of Silastic material. The peritoneal cavity is the preferred and most commonly utilized location for the shunt to terminate. There are two main advantages to placing the distal tubing in the peritoneum. First, if an infection develops, it usually stays localized rather than disseminating, as can happen with shunts placed in the heart. Second, a large amount of tubing can be placed in the peritoneal cavity to allow for growth of the child and minimize the need for revisions during expected childhood growth. In addition, the peritoneal cavity is an extremely efficient site of absorption and is also easily accessible to the surgeons. If the peritoneal cavity is not appropriate for placement of the distal tubing, either due to an abdominal malformation, postsurgical adhesions, infection, or inadequate reabsorption, the second and third choices for the distal catheter placement are the right atrium of the heart and the pleural cavity.
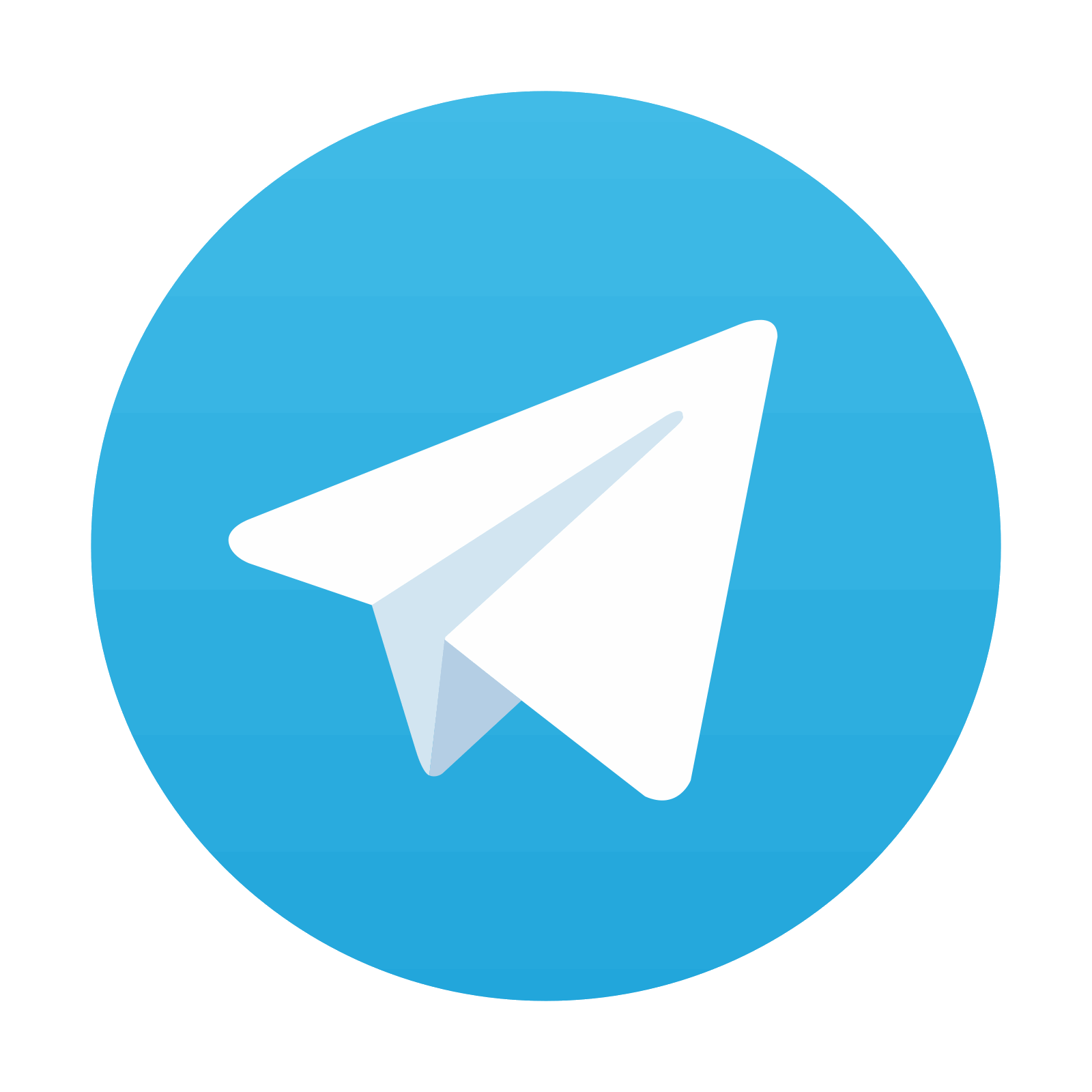
Stay updated, free articles. Join our Telegram channel
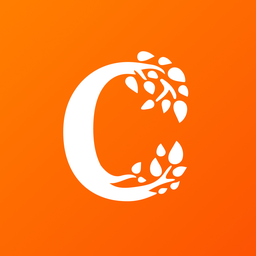
Full access? Get Clinical Tree
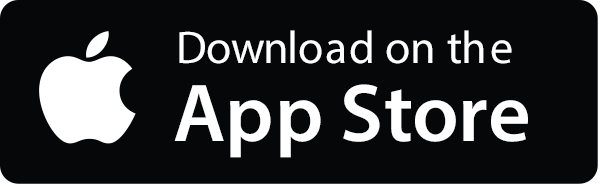
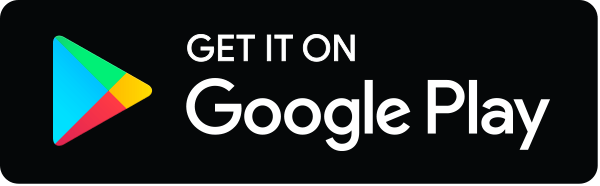