Common Neonatal Complications
Joan Renaud Smith
Annette Carley
Most newborns with complications are identified and cared for in community hospitals or level II perinatal centers (American Academy of Pediatrics [AAP], 2012). Perinatal nurses must have a thorough understanding of pathophysiologic and clinical signs of illness during the immediate newborn period. The length of stay limits the time to identify behavioral cues or subtle changes that could potentially compromise newborn well-being.
Conditions discussed in this chapter include common complications such as respiratory distress, congenital heart disease (CHD), hypoglycemia, hyperbilirubinemia, and sepsis. Less common but important topics include neonatal resuscitation, perinatal HIV-1 exposure, neonatal substance exposure, and hypoxic ischemic encephalopathy (HIE). The target population is term and preterm newborn infants, including the late preterm infant born between 34 0/7 and 36 6/7 weeks’ gestational age. This chapter concludes with a discussion of neonatal transport because, in some cases, the severity of the disease process necessitates transfer to a tertiary care center.
NEONATAL RESUSCITATION AND STABILIZATION
Most newborns transition from fetal to extrauterine life uneventfully. However, approximately 1 in 10 will require some assistance after delivery to initiate or sustain respiratory effort, and 1% will require extensive measures to survive. In keeping with the “ABCs” of resuscitation, providers must ensure that the airway is clear and unimpeded, breathing is spontaneous and unassisted, and that circulation is maintained to adequately perfuse tissues and organs. The AAP and the American Heart Association (AHA) (2011) recommend that all births be attended by someone capable of initiating resuscitation and those resources for sustained resuscitation efforts be available as needed.
Prior to birth, the fetus receives oxygen by diffusion from the mother’s blood across the placental membranes. Since the fetal lungs do not participate in oxygenation, only a small fraction of fetal blood passes through them. The fetal alveoli, although round and expanded, are fluid filled, and the surrounding arterioles are constricted. The increase in pulmonary vascular resistance (PVR) favors blood flow in a manner which bypasses the lungs through a series of fetal shunts, allowing delivery of optimally oxygenated blood through the ductus arteriosus to the body. After birth, the placenta no longer supports fetal needs, and the newborn must quickly establish ventilation, clear fluid from the alveoli, and dilate the pulmonary vasculature to support ongoing oxygenation. Failure to do so results in hypoxemia and acidosis. The newborn may respond briefly to hypoxia with compensatory tachypnea, although this is quickly followed by primary apnea and a fall in heart rate (HR). If breathing is not quickly established, secondary apnea occurs, and assisted ventilation must be provided to reverse the process (AAP & AHA, 2011).
Certain antepartum and intrapartum risk factors are associated with the need for resuscitation. Maternal factors may be chronic or acute and include such conditions as diabetes, hypertension, cardiopulmonary disease, substance exposure, late trimester bleeding, and infection. Intrapartum factors may also complicate fetal transition, including assisted delivery, cesarean delivery, abnormal fetal lie, presence of meconium, and placental complications. Newborns who are postterm, premature, or who have size-date
discrepancies pose additional risks for poor transition. An anticipated compromised birth warrants the presence of personnel who can initiate and sustain resuscitation, including use of ventilatory support, chest compressions, and selected medications. However, risk factors are not always apparent, and providers must be able to anticipate and intervene quickly to support the compromised newborn. Three assessment prompts will assist with quick identification of newborns who will require support: Is the baby term? Is the baby breathing? Is there good muscle tone? (AAP & AHA, 2011).
discrepancies pose additional risks for poor transition. An anticipated compromised birth warrants the presence of personnel who can initiate and sustain resuscitation, including use of ventilatory support, chest compressions, and selected medications. However, risk factors are not always apparent, and providers must be able to anticipate and intervene quickly to support the compromised newborn. Three assessment prompts will assist with quick identification of newborns who will require support: Is the baby term? Is the baby breathing? Is there good muscle tone? (AAP & AHA, 2011).
The Neonatal Resuscitation Program (NRP) was developed in 1987 to provide a systematic method for managing the compromised newborn. It supports consistent and appropriate actions to address ventilation and circulation needs, which are continually evaluated using an algorithm containing action blocks. The resuscitation sequence begins with positioning the newborn infant on his back or side to open the airway, and then proceeding to drying and stimulating. It is important to ensure resuscitation occurs in a warm environment. Following this initial 30-second block (“A”), the infant is assessed. If the infant responds with a sustained heart rate above 100 beats per minute and sustained breathing or crying, additional measures are unnecessary. However, an infant who does not establish sustained breathing is presumed to be exhibiting secondary apnea, and the next block (“B”) commences with assisted ventilation. The infant is continually assessed, and additional maneuvers are applied according to infant response (AAP & AHA, 2011).
Effective neonatal resuscitation for at-risk infants requires not only dexterity with maneuvers such as ventilation and compressions but also collaboration among a neonatal team to ensure timely and organized support. Poor communication and lack of teamwork have been identified as factors in poor outcomes following neonatal resuscitation, and the most recent edition updates recommendations to increase focus on team building and collaborating, and alternate learning strategies such as more effective use of simulation and debriefing (AAP & AHA, 2011; Perlman et al., 2010; Zaichkin & Weiner, 2011).
Some lingering controversies related to neonatal resuscitation and stabilization have prompted changes to the NRP guidelines, including the management of meconium exposure and the use of supplemental oxygen. Of the 13% of newborns born through meconium-stained amniotic fluid, less than 12% will go on to develop meconium aspiration syndrome (MAS). However, for many years, providers performed aggressive perineal and direct tracheal suctioning on all exposed infants in an attempt to prevent MAS. The latest recommendations for management of meconium-exposed infants include an assessment of infant behavior. For the meconiumexposed infant who is vigorous (e.g., with normal respiratory rate, tone, and HR), intrapartal suctioning and immediate neonatal tracheal suctioning are no longer recommended. However, for the meconiumexposed infant who is depressed, direct suctioning of the trachea before establishment of respirations is indicated (AAP & AHA, 2011; Bry, 2008).
Data regarding use of supplemental oxygen during neonatal resuscitation is evolving, and the ideal concentration of oxygen during resuscitation is unknown. Some evidence suggests enhanced risk for inadvertent oxidant injury across all gestational ages when supplemental oxygen is used. Until more definitive evidence is available, providers must attempt to avoid hypoxemia and hyperoxemia when supplemental oxygen is applied (Bry, 2008; Rabi, 2010), and the use of room air for initial resuscitation of the term infant is currently recognized as acceptable practice if well monitored. The most recent NRP guidelines recommend the use of pulse oximetry for neonatal resuscitations, especially of the preterm population, to more optimally titrate supplemental oxygen when used (Perlman et al., 2010; Zaichkin & Weiner, 2011).
An additional neonatal education program endorsed by the AAP is S.T.A.B.L.E., which reinforces key stabilization skills via an acronym: Sugar, Temperature, Airway, Blood pressure, Lab work assessment, and Emotional support of families. This program supports nursery staff who participate in postresuscitation stabilization and pretransport care of the neonate requiring intensive care and encourages a systematic approach to management (Taylor & Price-Douglas, 2008). Both S.T.A.B.L.E. and the NRP have been disseminated worldwide as stabilization programs for atrisk neonates.
RESPIRATORY DISTRESS
Respiratory distress is a major cause of neonatal morbidity and mortality despite significant technologic and pharmacologic advances during the past 30 years. Respiratory distress is one of the most common neonatal complications seen by the perinatal and neonatal nurse and is a principal indication for neonatal transfer to tertiary care units. The pathophysiology and etiology of respiratory distress varies, but the result is decreased ability to exchange the oxygen and carbon dioxide necessary to ensure delivery of well-oxygenated blood to vital organs. Respiratory distress may be an isolated finding or occur in association with other medical or systemic problems. It may be due to structural or functional abnormality or as a consequence of acute lung injury and result in prolonged transition to extrauterine life. Five of the most common respiratory diseases
occurring during the neonatal period are respiratory distress syndrome (RDS), MAS, pneumonia, transient tachypnea of the newborn (TTNB), and persistent pulmonary hypertension of the newborn (PPHN).
occurring during the neonatal period are respiratory distress syndrome (RDS), MAS, pneumonia, transient tachypnea of the newborn (TTNB), and persistent pulmonary hypertension of the newborn (PPHN).
RESPIRATORY DISTRESS SYNDROME
RDS primarily occurs in preterm newborns. In the United States, approximately 24,000 newborns develop RDS each year. The incidence of RDS is inversely related to gestational age: 60% of infants are born at less than 28 weeks, 30% of those are born at 28 to 34 weeks’ gestation, and less than 5% of those born after 34 weeks are affected (Warren & Anderson, 2009). The mortality rate for RDS across all gestational ages is about 10%, attributable to improved prenatal and postnatal management (Dudell & Stoll, 2007; Warren & Anderson, 2009). RDS is caused by insufficient amounts of surfactant or delayed or impaired surfactant synthesis. Surfactant is a mixture of phospholipids and proteins synthesized, packaged, and excreted by alveolar type II cells that lowers surface tension in the alveoli and functions as a stabilizer to prevent atelectasis and alveolar collapse at end expiration (Cole, Nogee, & Hamvas, 2006). Without surfactant, atelectasis (alveolar collapse) occurs, resulting in a series of events that progressively increase disease severity. These events include hypoxemia (decreased concentration of oxygen), hypercapnia (increased concentration of carbon dioxide), mismatch of ventilation with perfusion, acidosis, pulmonary vasoconstriction, alveolar endothelial and epithelial damage, and subsequent protein-rich interstitial and alveolar edema. This cascade of events further decreases surfactant synthesis, storage, and release and leads to pulmonary failure (Dudell & Stoll, 2007; Warren & Anderson, 2009).
MECONIUM ASPIRATION SYNDROME
Passage of meconium in utero or perinatally is primarily seen in term and postterm infants and those experiencing stress such as growth-restricted infants or those with cord complications compromising uteroplacental circulation (Dudell & Stoll, 2007). Meconium passage occurs as a response to hypoxia, as relaxation of the anal sphincter allows passive escape of meconium into the amniotic fluid. Under normal intrauterine conditions, amniotic fluid does not enter the fetal lung. However, when the fetus experiences hypoxemia, gasping may result in aspiration of meconium-stained amniotic fluid. Of newborns, 8% to 20% are exposed to amniotic fluid stained by meconium; of these, 5% to 10% will go on to develop MAS (American College of Obstetricians and Gynecologists [ACOG], 2006; Dudell & Stoll, 2007; van Lerland & de Beaufort, 2009).
Preventive strategies have been evaluated for cases at risk for MAS, including amnioinfusion and direct tracheal suctioning of the neonate. Although amnioinfusion appears to be a reasonable treatment for repetitive variable decelerations, its sole use as a technique to prevent MAS is not warranted (ACOG, 2006; Xu, Wei, & Fraser, 2008). When aspirated by the fetus before or during birth, meconium can obstruct the airways, leading to severe hypoxia, inflammation, and infection and cause significant respiratory difficulties. Past evidence suggested that intrapartum suctioning before the first breath would decrease the risk of MAS; however, subsequent evidence from a large multicentered randomized trial did not show benefit from routine intrapartum oropharyngeal and nasopharyngeal suctioning (Velaphi & Vidyasagar, 2006; van Lerland & de Beaufort, 2009). Currently, the NRP no longer recommends that all meconiumstained babies routinely receive intrapartum suctioning (AAP & AHA, 2011; Vain, Szyld, Prudent, & Aguilar, 2009).
Pneumonitis is an inflammatory response likely secondary to bile salts present in aspirated meconium. Pneumonitis results in acute lung injury with proteinrich interstitial and alveolar edema. In situations where meconium only partially obstructs the airway, a ball-valve effect results. Air enters the lower airways on inspiration but cannot escape on expiration. This causes overdistension of alveoli, leading to alveolar rupture and pulmonary air leaks. Pneumonitis and airway obstruction result in hypoxemia and acidosis, which cause increased PVR and subsequent PPHN (Steinhom & Farrow, 2007).
PNEUMONIA
Pneumonia is acquired through vertical or horizontal transmission of a pathogenic organism and may present clinically as early-onset sepsis develops in the neonate. Vertical transmission occurs in utero in association with chorioamnionitis, intraamniotic infection, transplacental transmission of organisms, or aspiration of infected amniotic fluid. It may also occur following prolonged rupture of the membranes due to loss of the bacteriostatic protection of amniotic fluid. Horizontal transmission occurs in the nursery as pathogenic organisms spread from hospital personnel, equipment, or families or present as secondary infections as the result of some other primary infection. Pneumonia causes an inflammatory process, disrupting the normal barrier function of the pulmonary endothelium and epithelium, leading to abnormal protein permeability and edema of lung tissue. Hypoxemia and acidosis result, causing increased PVR and potential sequelae such as PPHN (Stoll, 2007a).
TRANSIENT TACHYPNEA OF THE NEWBORN
TTNB occurs in approximately 0.3% to 0.5% of newborns, although the exact incidence is unknown
(Yurdakok, 2010). Generally, TTNB is a mild, selflimiting disorder of term and near-term infants (NTIs), lasting from 12 to greater than 72 hours. Fetal lungs are fluid filled during gestation, although production of lung fluid decreases at birth with the onset of breathing and secondary to other influences of labor. Fluid is then absorbed from the air spaces through blood vessels, lymphatics, and the upper airways (Yurdakok, 2010). In certain infants, however, the residual fluid in the alveoli persists, alters oxygen exchange, and increases work of breathing. Several pathophysiologic mechanisms have been suggested for TTNB. Historically, this condition was thought to be related to delayed reabsorption of lung fluid by the pulmonary lymphatic system. Retained fluid causes bronchiolar collapse with air trapping or hyperinflation of the alveoli. Hypoxia results when poorly ventilated alveoli are perfused, and hypercarbia results from mechanical interference with alveolar ventilation by fluid. Decreased lung compliance results in tachypnea and increased energy needed to do the work of breathing. It is known that stimuli during labor and at the time of birth cause active transport of chloride from plasma into the fetal lung fluid to cease. As the concentration of chloride becomes higher in the plasma, fetal lung fluid begins to be reabsorbed. Two thirds of the fetal lung fluid is absorbed before birth. Newborns without the benefit of labor and those born prematurely do not have the same amount of time to reabsorb lung fluid as those born after a normal course of labor. Infants delivered by elective cesarean section also have a higher incidence of TTNB (Jain & Eaton, 2006). Some also suggest that TTNB may result from mild immaturity of the surfactant system, which may explain cases of TTNB in late preterm infants (Yurdakok, 2010).
(Yurdakok, 2010). Generally, TTNB is a mild, selflimiting disorder of term and near-term infants (NTIs), lasting from 12 to greater than 72 hours. Fetal lungs are fluid filled during gestation, although production of lung fluid decreases at birth with the onset of breathing and secondary to other influences of labor. Fluid is then absorbed from the air spaces through blood vessels, lymphatics, and the upper airways (Yurdakok, 2010). In certain infants, however, the residual fluid in the alveoli persists, alters oxygen exchange, and increases work of breathing. Several pathophysiologic mechanisms have been suggested for TTNB. Historically, this condition was thought to be related to delayed reabsorption of lung fluid by the pulmonary lymphatic system. Retained fluid causes bronchiolar collapse with air trapping or hyperinflation of the alveoli. Hypoxia results when poorly ventilated alveoli are perfused, and hypercarbia results from mechanical interference with alveolar ventilation by fluid. Decreased lung compliance results in tachypnea and increased energy needed to do the work of breathing. It is known that stimuli during labor and at the time of birth cause active transport of chloride from plasma into the fetal lung fluid to cease. As the concentration of chloride becomes higher in the plasma, fetal lung fluid begins to be reabsorbed. Two thirds of the fetal lung fluid is absorbed before birth. Newborns without the benefit of labor and those born prematurely do not have the same amount of time to reabsorb lung fluid as those born after a normal course of labor. Infants delivered by elective cesarean section also have a higher incidence of TTNB (Jain & Eaton, 2006). Some also suggest that TTNB may result from mild immaturity of the surfactant system, which may explain cases of TTNB in late preterm infants (Yurdakok, 2010).
PERSISTENT PULMONARY HYPERTENSION OF THE NEWBORN
PPHN is defined as a failure of the pulmonary vasculature to relax at birth, resulting in delivery of unoxygenated blood to the systemic circulation. In fetal circulation, pulmonary blood vessels are constricted, causing most blood flow to bypass the lungs. This is appropriate for the fetus because the placenta rather than the fetal lung acts as the organ of gas exchange. PPHN is the result of a sustained elevation of the PVR after birth, preventing transition to the normal pattern of circulation. When the PVR remains elevated, blood bypasses the lungs by flowing through the foramen ovale or ductus arteriosus. This pattern of circulation is referred to as right-to-left shunting because blood is diverted from the venous circulation on the right side of the heart to the arterial circulation on the left side of the heart without going through the pulmonary vascular system. In approximately 1/500 to 1/1,500 live births, severe, prolonged hypoxemia (decreased oxygen in the blood) progresses to hypoxia (decreased oxygen in the tissues) and results in metabolic acidosis and worsening pulmonary vasoconstriction. A vicious cycle ensues. PPHN may be idiopathic, caused by abnormal development of pulmonary vessels, or may result from pathopysiologic events such as asphyxia, MAS, pneumonia, and RDS (Dudell & Stoll, 2007; Lapointe & Barrington, 2011; Stayer & Liu, 2010; Steinhom & Farrow, 2007). Infants with PPHN are typically labile and often require sedation to control competing respiratory effort, vasodilators to overcome pulmonary vasoconstriction, and vasopressors to support systemic blood pressure. A small percentage with refractory hypoxemia may require extracorporeal membrane oxygenation (ECMO) for survival (Dudell & Stoll, 2007).
ASSESSMENT OF RESPIRATORY DISTRESS
Clinical signs of respiratory distress may be present at birth or occur at any time in the early neonatal period. These signs include tachypnea, grunting, retractions, nasal flaring, and cyanosis. Tachypnea is defined as a sustained respiratory rate greater than 60 to 70 breaths per minute (Gardner, Enzman-Hines, & Dickey, 2011). Tachypnea develops when the newborn attempts to improve ventilation. Because of the very compliant chest wall, especially in the preterm newborn, it is more energy efficient for the newborn to increase the respiratory rate rather than the depth of respirations. However, persistent tachypnea results in muscular fatigue and, over time, further compromises pulmonary status.
On exhalation, a grunting sound is sometimes heard in newborns with respiratory distress. Grunting is the result of forceful closure of the glottis in an attempt to increase intrapulmonary pressure, keep alveoli open, and create residual lung gas volume (functional residual capacity). Keeping alveoli open during exhalation is a compensatory response to decreased partial pressure of oxygen (PO2) and allows more time for gas exchange to occur (Gardner et al., 2011).
Retractions are depressions observed between the ribs, above the sternum, or below the xiphoid process during inhalation. Retractions are the result of a very compliant chest wall and noncompliant lung. Compliance refers to the stiffness or distensibility of the chest wall and lung parenchyma. As the amount of negative intrathoracic pressure increases on inspiration, the rib cage expands until the soft tissue of the thorax and weak intercostal muscles are pulled inward toward the spine. The result is worsening atelectasis with marked oxygenation and ventilation abnormalities (Cifuentes, Segars, & Carlo, 2003; Gardner et al., 2011).
Nasal flaring occurs with respiratory distress as the newborn attempts to decrease airway resistance and increase the inflow of air through dilation of the alae nasi (Gardner et al., 2011).
Cyanosis results from inadequate oxygenation caused by atelectasis, poor lung compliance, and right-to-left shunting. Although the newborn’s color may be an indicator of oxygenation, variables such as skin temperature and perfusion affect the accuracy of this finding. Precise measurement of oxygen and acid-base status may be necessary for the management of respiratory distress using tools such as pulse oximetry and blood gases (Gardner et al., 2011; Rohan & Golembek, 2009).
INTERVENTIONS FOR RESPIRATORY DISTRESS
Care for newborns with respiratory distress focuses on oxygenation and ventilation as well as controlling factors that increase oxygen demands such as hypothermia or stress. Adequate oxygenation and ventilation requires supportive mechanisms ranging from supplemental oxygen only to application of assisted ventilation with techniques such as continuous positive airway pressure (CPAP) or mechanical ventilation. Pulse oximetry and direct arterial blood gas monitoring are methods used to ensure adequate gas exchange. In a preterm newborn, delivery of oxygen should be sufficient to maintain arterial oxygen tension at 50 to 70 mm Hg, corresponding to a pulse oximetry reading of approximately 85% to 95% (Dudell & Stoll, 2007). Because oxygen may be toxic to some tissue, care should be taken to avoid excessive tissue oxygenation, which might have toxic effects such as chronic lung disease or retinopathy of prematurity. In a term newborn at risk for PPHN, oxygen delivery should be sufficient to maintain normoxemia yet avoid hypoxia, which is a potent stimulus for vasoconstriction (Lapointe & Barrington, 2011). Infants with suspected PPHN will need to be transferred to a tertiary care center for further evaluation and management, including potential use of high-frequency ventilation (HFOV), inhaled nitric oxide (i-NO), or ECMO for severe hypoxemia (Stayer & Liu, 2010).
Select pharmacologic agents may be used in the prevention or management of neonatal respiratory distress. Prenatally, at-risk mothers may receive antenatal steroids to stimulate surfactant synthesis in an effort to prevent RDS. Postnatally, commonly used preparations include airway-instilled surfactant (for RDS prophylaxis or treatment), antibiotics (for pneumonia prophylaxis or treatment), and inhaled or vascularly delivered pulmonary vasodilators (for PPHN treatment) (Konduri & Kim, 2009; Warren & Anderson, 2009).
A neutral thermal environment (NTE) is crucial in the care of a newborn with respiratory distress. Hypothermia or hyperthermia both increase metabolic demands, leading to decreased oxygenation, metabolic acidosis, and worsening respiratory distress (Cifuentes et al., 2003). Newborns with respiratory distress are cared for under a radiant warmer or in an incubator.
Adequate nutrition frequently requires the administration of intravenous (IV) fluids during the early neonatal period. Care is taken to prevent hypoglycemia that may occur from respiratory distress and increased metabolic demands (Rohan & Golembek, 2009).
CONGENITAL HEART DISEASE
CARDIOVASCULAR SYSTEM
The cardiovascular system begins to develop in the third week of gestation and is fully developed by the end of the eighth week. It is the first major organ system to develop in the embryo. In the United States, an estimated 32,000 infants are expected to be affected with CHD annually. Of these, an approximate 25% require invasive treatment in the first year of life (AHA, 2012). Heart defects are among the most common birth defects and are the leading cause of birth defect-related deaths. However, the overall mortality has significantly declined over the past few decades (AHA, 2012). The cause of CHD cannot be ascribed to any single factor. Most cases are multifactorial, involving genetic predisposition, familial recurrence, and environmental factors. A family history of CHD is significant; if the mother has a history of a child with CHD, her risk of recurrence increases by threefold (Kenney, Hoover, Williams, & Iskersky, 2011). CHD can also be associated with chromosomal abnormalities (i.e., trisomies 21, 18, 13; chromosome deletion syndromes; DiGeorge deletion 22q; Turner syndrome; and Cornelia de Lange) and maternal-environmental factors, such as drugs (i.e., thalidomide, anticonvulsants, lithium, retinoic acid) and alcohol exposure, or diseases (e.g., insulin-dependent diabetes, maternal lupus erythematosus) and infections (e.g., rubella, Coxsackie B, and enteroviruses) (Kenney et al., 2011).
Cardiac lesions are classified as cyanotic, acyanotic, or according to the hemodynamic characteristics related to pulmonary blood flow. Five of the most commonly occurring cardiac lesions presenting in the early neonatal period include ventricular septal defect (VSD), tetralogy of Fallot (TOF), PDA, atrial septal defect (ASD), and d (dextro)-transposition of the great arteries (d-TGA).
The assessment to exclude CHD includes the following:
Close observation of cardiorespiratory status
Palpation of peripheral pulses
Blood pressures of the four extremities
Chest radiograph to evaluate heart size and pulmonary vascularity
Blood gas determinations to evaluate oxygenation and metabolic status
Evaluation of response to 100% oxygen (a hyperoxia test is used to differentiate respiratory disease from cyanotic heart disease)
The newborn with CHD or persistence of a fetal shunt may present shortly after birth or within the first weeks of life with cyanosis or symptoms of congestive heart failure (CHF). The newborn becomes cyanotic when gas exchange is impaired by pulmonary edema, blood flow to the lungs is restricted as a result of a structural abnormality, or blood flow is shunted away from the lungs. In the newborn with CHD, central cyanosis (bluish discoloration of the skin, nail beds, and mucous membranes) is one of the most common presenting signs and is generally not visible until there is 4 to 5 g/dL of deoxygenated hemoglobin in the arterial system (Mahle et al., 2009). Both the severity of hypoxemia and the hemoglobin concentration determine the degree of cyanosis (Kenney et al., 2011). It is important to differentiate central cyanosis from acrocyanosis (cyanosis of the extremities is commonly seen in newborns because of reduced blood flow through the small capillaries), which is considered a normal finding (Lott, 2007; Kenney et al., 2011).
When the heart is unable to meet the metabolic demands of the tissues, CHF ensues. Unlike infants with CHD, infants with CHF typically present with significant respiratory distress. Common clinical signs associated with CHF (Kenney et al., 2011; Lott, 2007):
Tachypnea (due to pulmonary edema)
Respiratory distress
Gallop rhythm (caused by dilation of the ventricles)
Decreased peripheral pulses and mottling of the extremities (decrease in peripheral tissue perfusion)
Tachycardia (in an attempt to compensate for a decrease in cardiac output [CO], the heart either increases the rate or the stroke volume [SV])
Hepatomegaly (right ventricle does not adequately empty, leading to an elevated right atrium pressure, resulting in hepatic venous congestion)
Poor feeding (due to high respiratory rate and increases in basal metabolic rate demands)
A murmur, if present, varies in quality and intensity, depending on the particular cardiac lesion present. If a VSD or ASD is present, allowing mixing of oxygenated and unoxygenated blood, only mild cyanosis occurs. If there is no intracardiac shunt, severe cyanosis is observed. With the exception of cyanosis, the physical examination is often otherwise unremarkable. In the newborn with a large VSD or ASD, signs of CHF develop over time as the PVR falls and the pulmonary blood flow increases. In newborns without an intracardiac shunt, severe hypoxemia and metabolic acidosis develop, followed by a rapid demise if emergency measures are not instituted.
Routine newborn screening for critical congenital heart disease (CCHD) using pulse oximetry is recommended to prevent mortality and morbidity (Secretary’s Advisory Committee on Heritable Disorders in Newborns and Children, 2011). Hypoplastic left heart syndrome, pulmonary atresia (with intact septum), transposition of the great arteries, truncus arteriosus, tricuspid atresia, tetralogy of Fallot, and total anomalous pulmonary venous return are among the seven CCHDs that are primary targets for the routine screening in the well infant and intermediate nurseries (Mahle et al., 2009; Kemper et al., 2012).
VENTRICULAR SEPTAL DEFECT
Pathophysiology
The partitioning of the embryonic heart into chambers of the atria and ventricles begins near the fourth week of gestation and is completed by the end of the seventh week (Auckland, 2010). A VSD is present when there is an incomplete division of the right and left ventricles. VSDs are classified by their anatomic location; perimembranous and muscular are the two most common types. A perimembranous VSD is located just below the aortic valve and accounts for 80% of all VSDs. A muscular VSD is located in the muscular septum. Of membranous and muscular VSDs, 75% to 80% close spontaneously. A VSD is considered an acyanotic lesion with increased pulmonary blood flow. The size and location of the defect, as well as the pulmonary-tosystemic vascular resistance ratio, determine the degree of left-to-right shunt. The timing of the onset of symptoms is directly related to the normal fall in the PVR after birth (Kenney et al., 2011).
Assessment
The onset of symptoms resulting from a VSD is related to the size of the defect and PVR. A newborn with a small defect has minimal left-to-right shunting at the ventricular level and may appear well with few or no symptoms other than a holosystolic systolic murmur heard best at the lower left sternal border. The murmur develops as the PVR falls. A newborn with a large defect may present with symptoms of CHF but not until approximately 2 to 4 weeks of life. As with the smaller defects, the murmur is holosystolic and heard over the left lower sternal border. Preterm newborns with large VSDs may present sooner and be more symptomatic compared to their term counterparts because preterm infants have lower PVR at birth, resulting in greater left-to-right shunting (Kenney et al., 2011).
TETRALOGY OF FALLOT
Pathophysiology
TOF consists of a large perimembranous VSD, pulmonary artery stenosis, an overriding aorta, and right ventricle hypertrophy (Kenney et al., 2011). This lesion is a result of disordered embryonic cardiac functioning. TOF occurs during the embryonic stage of development, when some unknown factor influences
functioning of the heart at the cellular level. This alteration in cellular function is partly responsible for determining development. TOF is generally considered a cyanotic lesion with decreased pulmonary blood flow, but the hemodynamics vary widely, depending on the severity of pulmonary stenosis, the size of the VSD, and the pulmonary and systemic vascular resistance. Most newborns with TOF present with cyanosis because of the right-to-left intracardiac shunt. However, if the intracardiac shunt is mainly left to right due to a mild or moderate right ventricular outflow obstruction, the infant will not be cyanotic (Kenney et al., 2011). Typically, the course worsens over the first year of life.
functioning of the heart at the cellular level. This alteration in cellular function is partly responsible for determining development. TOF is generally considered a cyanotic lesion with decreased pulmonary blood flow, but the hemodynamics vary widely, depending on the severity of pulmonary stenosis, the size of the VSD, and the pulmonary and systemic vascular resistance. Most newborns with TOF present with cyanosis because of the right-to-left intracardiac shunt. However, if the intracardiac shunt is mainly left to right due to a mild or moderate right ventricular outflow obstruction, the infant will not be cyanotic (Kenney et al., 2011). Typically, the course worsens over the first year of life.
Assessment
TOF is the most common cyanotic heart disease seen in the first year of life. Newborns with TOF are most often diagnosed in the first few weeks of life due to either a loud murmur or cyanosis. Newborns with TOF often present with cyanosis, hypoxia, and dyspnea. However, newborns who are symptomatic typically have severe right ventricular outflow tract obstruction (Kenney et al., 2011). The timing and degree of cyanosis depend on the severity of the pulmonary stenosis and may not be noticed until closure of the ductus arteriosus. In the case of pulmonary atresia and hypoplasia of the pulmonary arteries, marked cyanosis may be observed immediately after birth. The clinical signs of right-sided heart failure, resulting from right ventricular outflow tract obstruction, include hepatomegaly, tricuspid valve regurgitation, and a grade II to IV/VI harsh systolic murmur best heard over the mid to upper left sternal border.
PATENT DUCTUS ARTERIOSUS
Pathophysiology
The ductus arteriosus is a normal pathway of fetal circulation. The ductus arteriosus connects the pulmonary artery to the aorta, allowing blood to bypass the lungs directly into the placenta. During fetal life, PVR is greater than systemic vascular resistance. After birth, with spontaneous respiration, the arterial oxygen level increases and PVR decreases, causing the ductus to close. Functionally, the PDA closes within hours to several days after birth, but closure is often delayed in premature infants. If the ductus arteriosus does not close, blood begins to flow left to right through the patent ductus as the PVR decreases. A PDA is an acyanotic lesion with increased pulmonary blood flow. It presents with signs and symptoms of CHF. It occurs much more commonly in preterm newborns, with the incidence inversely proportional to gestational age (Kenney et al., 2011).
Assessment
The manifestation of PDA depends on the gestational age and the degree of lung disease. Preterm newborns generally develop signs associated with CHF at 3 to 7 days of life, but it can develop sooner in the smaller preterm newborn treated with surfactant. The development of clinical signs is related to the normal fall in the PVR, resulting in increased blood flow to the pulmonary circulation and volume overload of the left ventricles. In newborns, a grade I through III systolic ejection murmur will likely develop; if left untreated, a classic machinerylike continuous murmur may result in older infants and children. PDA murmurs are best heard at the upper left sternal border (over the first and second intercostal spaces to the left of the sternum) and may radiate to the back, between the scapulae. However, with a right-to-left shunt, a murmur may be absent (Kenney et al., 2011).
ATRIAL SEPTAL DEFECT
Pathophysiology
The separation of the atrium begins near the middle of the fourth week of gestation and is completed by the sixth week, leaving the foramen ovale open between the two atria. An abnormality occurring during atrial separation can result in an ASD. An ASD is considered an acyanotic lesion with increased pulmonary blood flow. Approximately 10% of newborns with very large ASDs develop CHF as the PVR decreases and a left-toright shunt develops with concomitant right ventricular volume overload and hypertrophy (Sadowski, 2010). Three major types of ASDs occur and are differentiated from each other by whether they involve other structures of the heart and how they are formed during fetal development (Lott, 2007). The first type is ostium secundum, the most common yet least serious type of ASD, and is caused when a part of the atrial septum fails to close completely while the heart is developing. The second type is an ostium primum defect, part of the spectrum of atrioventricular (AV) canal defects, which is often associated with a cleft in the leaflet of the mitral valve. The third type is the sinus venosus defect, which occurs at the superior vena cava and right atrium junction and is most often associated with partial anomalous pulmonary venous connection.
Assessment
Newborns with an uncomplicated ASD are generally asymptomatic. However, about 10% present with signs of CHF, poor feeding, and poor growth. These symptoms develop as the PVR falls over the first few weeks of life. Associated with an ASD is a soft, systolic murmur best heard over the second intercostal space at the left upper sternal border.
d-TRANSPOSITION OF THE GREAT ARTERIES
Pathophysiology
The truncus arteriosus begins to divide during the fifth week of gestation. As the cardiac tube folds, the vessel twists on itself and divides into two separate vessels. The exact etiology of transposition remains unknown. Historically, transposition was thought to occur because of a failure of the aorticopulmonary septum to grow in a spiral fashion, resulting in inappropriate migration of the vessels. However, additional causes continue to be explored (Sankaran & Brown, 2007). The d (dextro)-transposition of the great arteries (d-TGA) occurs when the aorta arises from the right ventricle and the pulmonary artery arises from the left ventricle, resulting in pulmonary and systemic circulations functioning in parallel. When these two arteries are transposed, unoxygenated blood returning from the body enters the right side of the heart and returns to the body, and oxygenated blood returning from the lung enters the left side of the heart and returns to the lungs. d-TGA is considered a cyanotic lesion with increased pulmonary blood flow. d-Transposition can occur in isolation or can be associated with other defects (e.g., PDA, ASD, VSD, pulmonary stenosis). The degree of cyanosis depends on the amount of mixing of oxygenated and unoxygenated blood between the parallel systemic and pulmonary circulations through the associated lesions (e.g., patent foramen ovale [PFO], VSD, ASD, PDA, or collateral circulation) (Kenney et al., 2011).
Assessment
d-TGA is the most common cyanotic heart lesion that presents in the newborn period and is more prevalent in males (Kenney et al., 2011). The newborn with d-TGA presents with cyanosis typically within the first hours of life, and the degree of cyanosis varies depending on the amount of intracardiac mixing. For instance, if the mixing occurs through a large VSD or PDA, the cyanosis may be mild. If the ventricular septum is intact or the PDA is closing, the cyanosis is profound since there is no intracardiac shunt. With the exception of cyanosis, the physical examination findings are often otherwise unremarkable. With a large VSD or ASD, signs of CHF develop over time as the PVR falls and the pulmonary blood flow increases. In the absence of an intracardiac shunt, severe hypoxemia and metabolic acidosis develop, followed by a rapid demise if emergency measures are not instituted.
INTERVENTIONS FOR CONGENITAL HEART DISEASE
Newborns with known or suspected CHD usually require transfer to a tertiary center for treatment and follow-up. The complete diagnostic workup and subsequent repairs or palliative surgery are performed in centers with pediatric cardiac capabilities. Before transport, close observation and supportive care and treatment are warranted. Nursing care for newborns with known or suspected CHD includes the following:
Cardiorespiratory monitoring
Pulse oximetry
Blood work, including blood gas determinations
Ongoing assessment of color, perfusion, and degree of respiratory distress
Maintaining a neutral thermal environment
IV hydration and nutrition
Oxygen therapy, if appropriate, and mechanical ventilation, if required
Metabolic acidosis is treated with sodium bicarbonate, pulmonary edema with respiratory distress is treated with diuretics, and shock is treated with vasopressors and calcium gluconate. A lesion such as d-TGA without an intracardiac shunt is treated with prostaglandin E1 to maintain patency of the ductus arteriosus until surgical correction takes place (Kenney et al., 2011).
HYPOGLYCEMIA
During the neonatal period, transient low glucose levels are not only common but also likely a normal adaptation to extrauterine life (Williams, 2005). Blood glucose as low as 30 mg/dL may occur during the first hours following birth (Committee on Fetus and Newborn & Adamkin, 2011). One of the major difficulties associated with defining hypoglycemia is the lack of correlation between a given blood glucose level and clinical signs. Whether producing symptoms or asymptomatic, hypoglycemia can result in either normal neonatal outcome or serious neurologic sequelae, such as brain injury, learning disabilities, and cerebral palsy. The clinical effects of hypoglycemia remain poorly defined. Neuropathology is available in only a few infants who have died after severe hypoglycemia, although follow-up studies of high-risk infants suggest that adverse neurodevelopmental outcomes are more prevalent when there is a history of asymptomatic hypoglycemic in the newborn period (McGowan & Perlman, 2006). Although the exact incidence is elusive due to inconsistent definitions, hypoglycemia is estimated to occur in 1 to 3/1,000 live births and up to 15% of those who are born growth restricted (McGowan, Rozance, Price-Douglas, & Hay, 2011; Stoll, 2007b).
Rather than identifying strict definitions of hypoglycemia, most authors suggest the use of operational thresholds. There is no absolute threshold applicable to all babies, and there is no glucose concentration that absolutely determines clinical risk or predicts sequelae. A glucose value must be assessed in conjunction with other clinical data, and treatment should be based upon this integrated input (Cornblath et al., 2000).
PATHOPHYSIOLOGY
During fetal life, insulin is secreted by the fetal pancreas in response to glucose that readily crosses the placenta. At birth, the newborn’s blood glucose level is approximately 70% to 80% that of the mother. After removal of placental circulation, the newborn must maintain glucose homeostasis. This requires initiation of various metabolic processes, including gluconeogenesis (e.g., forming glucose from noncarbohydrate sources such as protein and fat) and glycogenolysis (e.g., conversion of glycogen stores to glucose), as well as an intact regulatory mechanism and an adequate supply of substrate (Kayiran & Gurakan, 2010; Sperling & Menon, 2004). While this is effective for the well or term infant, the sick or preterm infant is constrained in effectively mobilizing or utilizing fuel sources.
Hypoglycemia can occur at variable times in neonatal life, depending on its cause. Hypoglycemia during the first few hours of life can be a transient result of developmental immaturity or perinatal stress and may occur in preterm or small-for-gestational-age (SGA) infants. Beyond the first few hours of life, hypoglycemia is more common due to hyperinsulinemia, as with the infant of a diabetic mother. Persistent hypoglycemia, a rare event, may represent inborn metabolic errors of metabolism or endocrine disorders (Sperling & Menon, 2004).
Early feeding will contribute to stabilization of newborn blood sugar. Breastfed term infants have lower blood glucose levels but higher concentrations of ketone bodies than formula-fed infants (Hawdon, Ward Platt, & Aynsley-Green, 1992). Utilization of this alternate fuel source may allow them to tolerate lower serum glucose levels without sequelae (Committee on Fetus and Newborn & Adamkin, 2011; Cornblath et al., 2000).
ASSESSMENT
Identification of those infants at risk for developing hypoglycemia facilitates planning and implementation of appropriate nursing care. This process begins with a review of maternal prenatal and intrapartum history for risk factors associated with neonatal hypoglycemia and a careful physical examination. Symptoms of hypoglycemia are nonspecific and not easily differentiated from many other common neonatal conditions (Display 21-1).
Universal blood glucose screening before clinical signs develop is not currently recommended by the AAP (Committee on Fetus and Newborn & Adamkin, 2011). Selective screening of at-risk newborns is more appropriate and does not appear to decrease quality of care or result in adverse outcomes.
Newborns at risk should be screened within 30 to 60 minutes after birth. Use of proper screening techniques is one of the most important nursing functions. Point-of-care testing (POCT), performed using a bedside glucose oxidase stick method, will provide an expedient estimation of glucose values. Although glucose oxidase sticks are widely used, accuracy of the results from these screening tests depends on the hematocrit, blood source, and operator’s skill. They have been shown to have considerable variance from actual blood glucose levels, which may be due to the source of blood used for testing. Venous blood samples have glucose levels that are approximately 10% less than capillary or arterial specimens (Deshpande & Ward Platt, 2005). Other factors limiting accuracy are improper storage, outdated shelf life of test strips, or contamination with isopropyl alcohol, which falsely elevates the glucose result. It is also important to remember that these POCT use whole blood, which will affect the ability to accurately detect glucose at extremes of hematocrit. The timing of glucose measurements may also result in inaccurate values, as failure to run the test promptly after sampling may result in red blood cell (RBC) oxidation of glucose and produce falsely low values. Blood samples should be transported on ice and analyzed quickly.

Jitteriness, tremors
Tachypnea, grunting
Diaphoresis
Cyanosis
Lethargy
Hypotonia
Irritability
Temperature instability
Apnea
Seizures, coma
INTERVENTIONS
Newborns with asymptomatic hypoglycemia should be fed immediately and then retested. Invasive interventions on the basis of low values detected by screening are not warranted as long as infants are assessed and are found to be without clinical findings attributable to hypoglycemia. A low glucose in the asymptomatic newborn may initially be managed by offering breastfeeding or providing expressed breast milk or formula. The infant should be reassessed within 2 to 4 hours and, if the glucose remains low for a second measurement, should be fed again or have IV therapy started (Cornblath et al., 2000).
Newborns with symptomatic hypoglycemia, particularly those with neurologic signs and low POCT bedside blood glucose, should be treated immediately with an IV infusion of glucose, and a blood sample should be drawn and sent to the laboratory for glucose evaluation. Infusion rates should be similar to that expected with endogenous hepatic glucose production (approximately 5 mg/kg/min depending on maturity and weight for gestation—equivalent to 10% dextrose at approximately 70 to 80 mL/kg/day or 3 mL/kg/hr and titrated based on response) (Williams, 2005). Gradual increases in glucose infusion rate should not exceed 2 mg/kg/min each hour. Newborns who are unable to nipple feed and those whose blood glucose levels do not respond to oral feedings or have very low glucose levels (e.g., < 20 mg/dL) should receive a 200 mg/kg (2 mL/kg) bolus of 10% dextrose in water intravenously over 1 minute, followed by a continuous infusion at the rates given previously until the blood glucose is stabilized (Cornblath et al., 2000; Rozance & Hay, 2010). Correction of hypoglycemia should result in resolution of the symptoms. IV administration is tapered off slowly, and the blood glucose level is monitored frequently, every 1 to 2 hours initially, and then intermittently before feedings until stable (Williams, 2005).
Newborns who experience persistent hypoglycemia may require an increased concentration of glucose, such as 12.5%, 15%, or 20%; dextrose solutions with concentrations greater than 12.5% require placement of a central line because of the risk of tissue extravasation. Other treatments for persistent or refractory hypoglycemia include glucagon, which promotes glycogenolysis and requires adequate stores, and corticosteroids, which induce gluconeogenic enzyme activity (Jain et al., 2010).
Hypoglycemia severe enough to warrant IV therapy, or which persists or recurs, requires further investigation to rule out underlying pathology, particularly infection or metabolic and endocrine disease (Deshpande & Ward Platt, 2005). The focus of nursing care is to prevent hypoglycemia when possible. Newborns should be fed within the first 2 hours of life. Care is taken to avoid cold stress and to recognize signs of respiratory distress and sepsis, which can increase the newborn’s risk for developing hypoglycemia.
HYPERBILIRUBINEMIA
Hyperbilirubinemia resulting in clinical jaundice is detected in up to 60% of term and in 80% of preterm newborns (Juretschke, 2005; Piazza & Stoll, 2007). Typically, healthy newborns are discharged from the hospital before the usual peak of total serum bilirubin (TSB) (72 to 120 hours). Most jaundice is benign and resolves within 7 to 10 days in term newborns. However, severe hyperbilirubinemia may develop in 8% to 9% of all newborns during the first postnatal week (Kamath, Thilo, & Hernandez, 2011). Jaundice is a common indication for hospital readmission, affecting 1 in 100 term or late preterm infants (Alkalay, Bresee, & Simmons, 2010). Because of the potential for bilirubin toxicity, newborns require assessment to identify those at risk for severe hyperbilirubinemia or, in rare cases, bilirubin encephalopathy or kernicterus. Unconjugated hyperbilirubinemia results from physiologic mechanisms (Display 21-2) or pathologic causes (Display 21-3).

Increased bilirubin related to relative polycythemia and short (80- to 90-day) life span of fetal red blood cells
Decreased uptake of bilirubin by the liver
Decreased enzyme activity and ability to conjugate bilirubin
Decreased ability to excrete bilirubin
Breastfeeding
PATHOPHYSIOLOGY
Bilirubin is produced from the breakdown of heme-containing proteins (Juretschke, 2005). The major hemecontaining protein is hemoglobin, which is the source of approximately 75% of the bilirubin produced. Heme is acted on by the enzyme heme oxygenase, releasing carbon monoxide and biliverdin. Biliverdin is then reduced to bilirubin through the activity of the enzyme biliverdin reductase. The degradation of every 1 g of hemoglobin produces 34 to 35 mg of bilirubin. Bilirubin binds with albumin for transport to the liver. Bilirubin, but not albumin, diffuses into the liver
cytoplasm, where it is transported to the endoplasmic reticulum for conjugation. Bilirubin combines with glucuronate with the help of glucuronyl transferase, the conjugating enzyme. Conjugated bilirubin is water soluble and excreted into bile and subsequently into the small intestine through the common bile duct. In the gut, conjugated bilirubin is excreted from the body through stool or converted to unconjugated bilirubin by a gut enzyme (beta-glucuronidase) that renders it reabsorbable. In fetal life, this reabsorption facilitates transport of bilirubin to the placenta for maternal excretion; however, postnatally, this pathway adds to the infant’s bilirubin load (Kamath et al., 2011; Piazza & Stoll, 2007; Thilo, 2005).
cytoplasm, where it is transported to the endoplasmic reticulum for conjugation. Bilirubin combines with glucuronate with the help of glucuronyl transferase, the conjugating enzyme. Conjugated bilirubin is water soluble and excreted into bile and subsequently into the small intestine through the common bile duct. In the gut, conjugated bilirubin is excreted from the body through stool or converted to unconjugated bilirubin by a gut enzyme (beta-glucuronidase) that renders it reabsorbable. In fetal life, this reabsorption facilitates transport of bilirubin to the placenta for maternal excretion; however, postnatally, this pathway adds to the infant’s bilirubin load (Kamath et al., 2011; Piazza & Stoll, 2007; Thilo, 2005).

Hemolytic disease of the newborn
Bruising, extravascular blood
Polycythemia
Intestinal obstruction
Metabolic conditions
Prematurity
Infection
Respiratory distress
Excretion of conjugated bilirubin is facilitated by bacteria in the gut. Meconium contains large amounts of bilirubin, but excretion is inhibited in the newborn because of the sterility of the gut. Normal colonization of bacteria occurs over time and is facilitated by early and frequent feeding. Feeding introduces bacteria into the gut. Lack of bacterial flora allows conversion of conjugated bilirubin back to an unconjugated form. This, along with greater red cell mass per kilogram in the newborn than in the adult and a shortened red cell life span, sets the stage for development of physiologic unconjugated hyperbilirubinemia. Newborns produce twice as much bilirubin as adults (Halamek & Stevenson, 2002; Piazza & Stoll, 2007; Thilo, 2005).
In a term newborn, physiologic unconjugated hyperbilirubinemia is characterized by a progressive increase in serum bilirubin to a peak of 6 to 8 mg/dL at 72 hours of age and a steady decline over the next week. In a preterm newborn, bilirubin continues to rise until the fourth to seventh postnatal day, reaching a peak of 8 to 12 mg/dL and decreasing thereafter as the processes of metabolism and excretion mature (Halamek & Stevenson, 2002). When jaundice is evident within the first 24 to 36 hours of life, bilirubin levels rise > 5 mg/dL/day or peak in excess of 12 to 14 mg/dL; or if jaundice persists beyond 2 weeks of life, it is less likely to represent a physiologic process and warrants assessment (Burgos, Flaherman, & Newman, 2011; Piazza & Stoll, 2007).
Hyperbilirubinemia may result from three mechanisms: increased bilirubin production, increased bilirubin reabsorption, or decreased bilirubin excretion and may be attributed to physiologic or pathologic causes. Conditions contributing to physiologic, unconjugated hyperbilirubinemia include normal bilirubin load from a large fetal RBC mass with a shortened life span, as well as delayed stooling. Breastfed infants may experience exaggerated jaundice related to initial decreased caloric intake, decreased stooling with subsequent increase of enterohepatic circulation, or effects of substances within the milk, which interfere with conjugation and excretion (Kamath et al., 2011
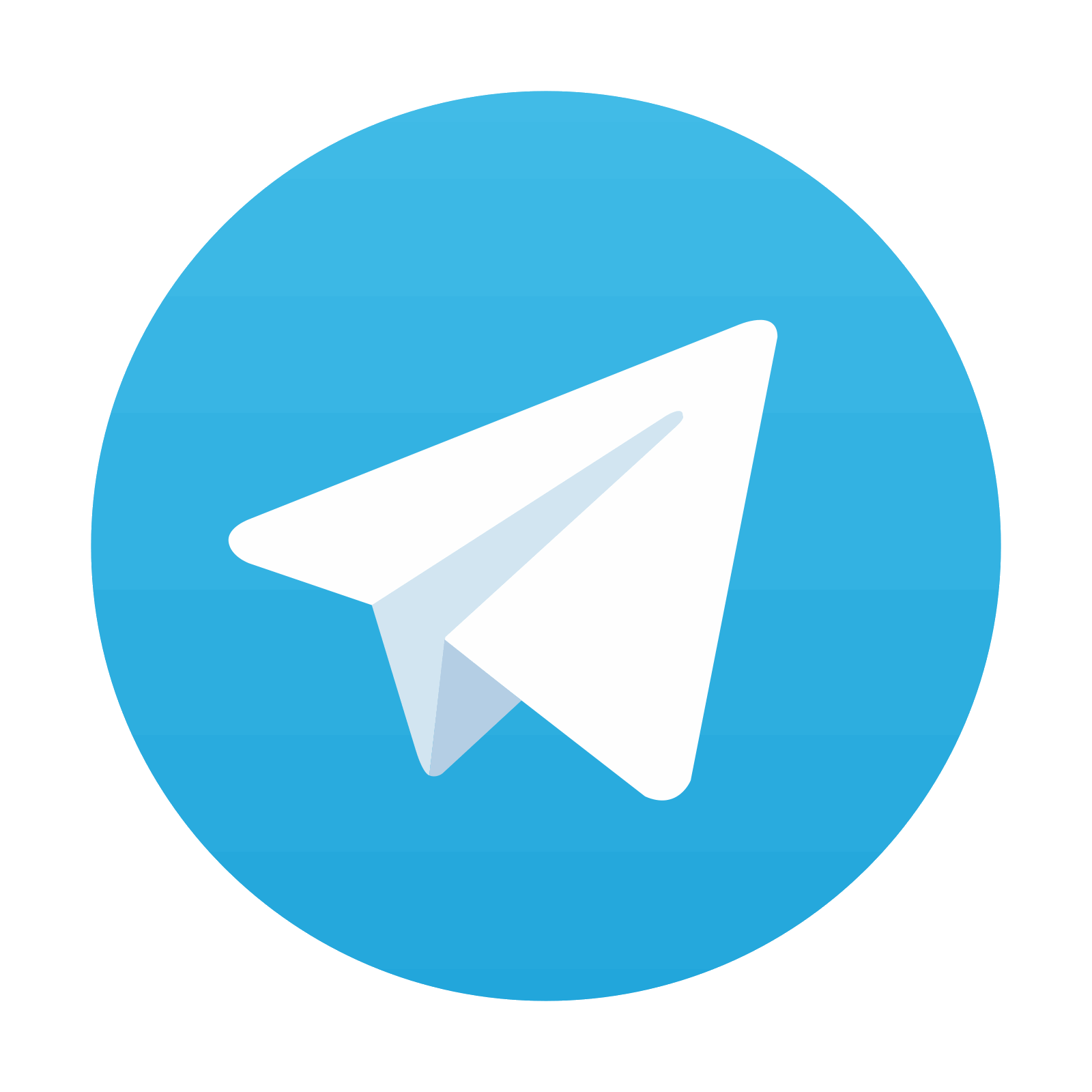
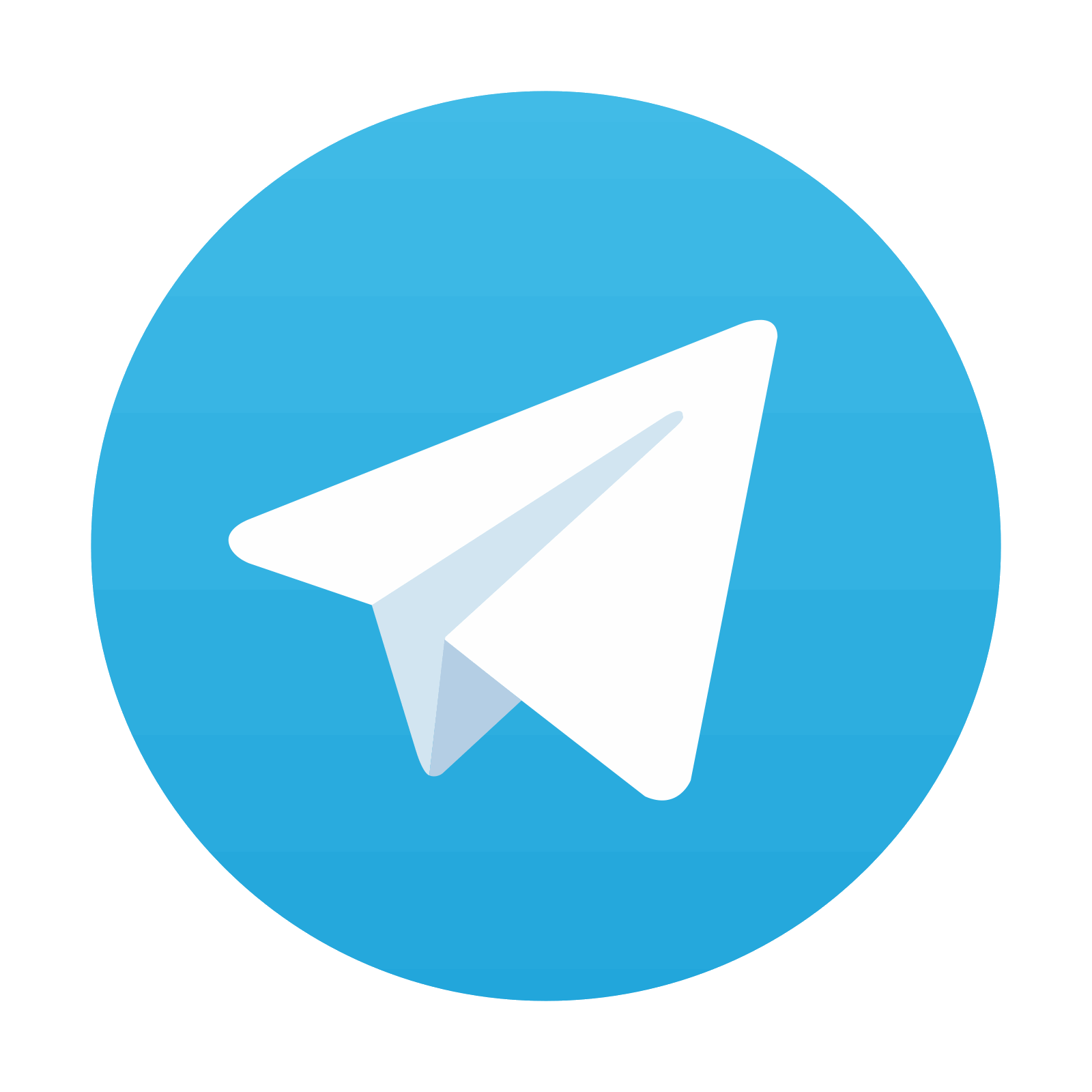
Stay updated, free articles. Join our Telegram channel
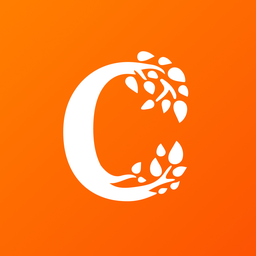
Full access? Get Clinical Tree
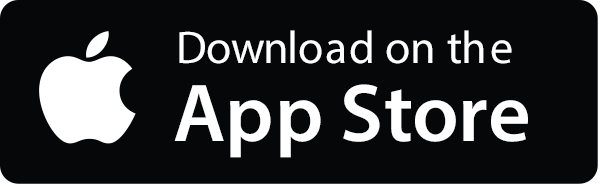
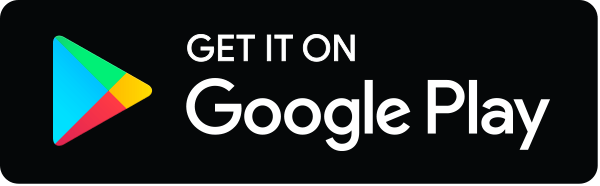