- Definition of blood gas parameters
- Principles of oxygen and carbon dioxide exchange in the lungs, and blood transport of these gases around the body
- Defining pH, acid, base and buffer
- Principles of acid-base homeostasis (maintaining normal blood pH)
- Defining the four types of acid-base disturbance
- respiratory acidosis and respiratory alkalosis
- metabolic acidosis and metabolic alkalosis
- respiratory acidosis and respiratory alkalosis
- Causes and consequences of acid-base disturbance
- Causes and consequences of hypoxaemia and hyperoxaemia
- Assessing blood oxygenation (blood gases v pulse oximetry)
- Principles of sampling arterial blood
- Foetal pH monitoring
The use of the term blood gases does not fully describe this test because although it includes measurement of the two significant gases dissolved in blood, oxygen (O2) and carbon dioxide (CO2), it also includes measurement of the pH of blood and several other parameters that are calculated from these measurements. In broad terms the test allows assessment of two related physiological functions: the facility of the lungs to simultaneously add oxygen to blood and remove carbon dioxide from blood (the dual process called pulmonary gas exchange); and the ability of the body to maintain the pH of blood within narrow healthy limits (called acid-base balance or acid-base homeostasis). The test is used for monitoring two patient groups in whom either one or both of these physiological functions may be disturbed: the critically/acutely ill and those with chronic respiratory disease. Clinically significant changes in the measured parameters of blood gases can occur over very short periods of time in the critically ill, so that patients may require blood gas measurement every few hours. For this reason blood gas analysers are often sited where critically ill patients are being cared for, in intensive care units, emergency departments and recovery rooms. In these circumstances responsibility for blood gas analysis often falls upon nursing staff. It is the only blood test that requires sampling of arterial blood; all other blood tests are performed on venous blood. Strictly speaking then, the test should be, and often is, called arterial blood gases.
Normal physiology
Normal cellular metabolism is associated with continuous production of carbon dioxide (CO2) and hydrogen ions (H+), as oxygen (O2) is consumed. The rates of production and consumption vary according to the level of metabolic activity. Health demands that despite this variation in production and consumption, the blood content of all three be maintained within narrow limits. The mechanism that maintains the three parameters within normal limits is a complex synergy of action involving chemical buffers in blood, the red cells (erythrocytes) that circulate in blood and the function of three organs: lungs, kidney and brain. Blood gases is a test that monitors the ability of the body to maintain these mechanisms. An understanding of test results then depends on a basic knowledge of respiratory physiology and normal acid-base balance. Although interrelated, these two topics are treated separately here for convenience only.
Respiratory physiology
Oxygen is fundamental to life. The cells of all human tissues derive the energy they require to survive and function from the continuous aerobic metabolism of dietary derived nutrients (carbohydrates, fats etc.). This aerobic metabolism requires a constant supply of oxygen and results in continuous production of carbon dioxide, a waste product that must be eliminated from the body. The object of respiration is to supply oxygen, present in inspired air to every tissue cell and eliminate the carbon dioxide these cells produce in expired air. Venous blood returning to the heart from the tissues is low in oxygen and loaded with carbon dioxide (see Figure 7.1). It is mixed in the right side of the heart and pumped to the lungs via the pulmonary artery. In the lungs, carbon dioxide passes from blood in exchange for oxygen. The blood, now with less carbon dioxide but loaded with oxygen, is pumped back to the heart via the pulmonary vein and out, via the aorta through the arterial system, for delivery of oxygen to the tissues.
Basic principles of gases in biological systems: units of measurement and diffusion
The amount of a gas present in systems, including biological systems is defined by the pressure it exerts, traditionally measured as the height in millimetres (mm) of a column of mercury (Hg). For example, the pressure of atmospheric air (i.e. barometric pressure) at sea level is 760 mmHg. This means that at sea level, the gases contained in the air we breathe have a combined pressure sufficient to support a column of mercury 760 mm high. In a mixture of gases, as air is, the total pressure is simply the sum of the partial pressures (represented by the symbol P) of each gas. So that since air comprises 21% oxygen, 0.03% carbon dioxide and 78% nitrogen, the partial pressure of oxygen (PO2) in inspired air at sea level is equal to 21% of total atmospheric pressure (i.e. 21/100 × 760) or 150 mmHg and partial pressure of carbon dioxide (PCO2) = 0.03/100 × 760 or 0.2 mmHg.
Figure 7.1 Oxygen and carbon dioxide content of air within lungs, systemic blood (venous and arterial) and tissues.
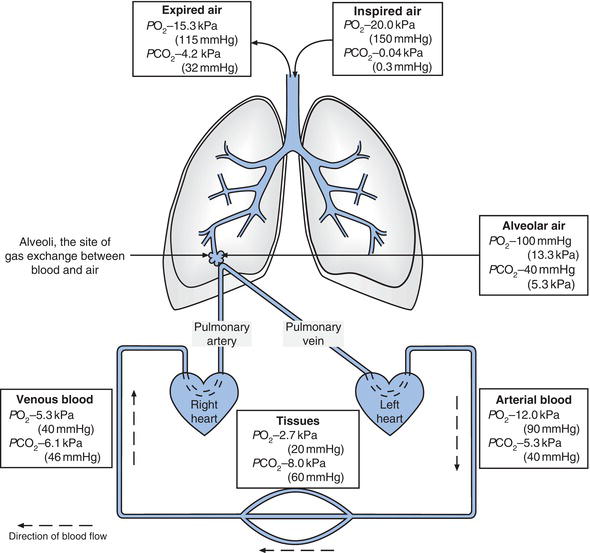
In clinical laboratories, the Systeme Internationale (SI) unit of pressure, the kilo-Pascal (kPa), has replaced mmHg as the unit of choice when measuring partial pressures of gases. Pressure is defined as force per unit area. The SI unit of force is the Newton (N) and the SI unit of area is the square metre (m2). Thus the derived SI unit of pressure, the Pascal (named after the seventeenth-century physicist), is defined as 1 Newton per square metre (1N/m2). The kilo-Pascal (kPa) is one thousand Pascals (i.e. 1000 N/m2). Some physiology texts continue to express the partial pressure of gases in blood in mmHg. To convert mmHg to kPa, simply multiply by 0.133. Figure 7.1 describes the PO2 and PCO2 of inspired air, alveolar air (the air deep within the lungs), venous blood, arterial blood and tissues.
The rate of diffusion of a gas across a physiological membrane is determined by the partial pressure of that gas on either side of the membrane. Gas diffuses from high partial pressure to low partial pressure. The greater the difference on either side of the membrane, the faster gas diffuses. The significance of this simple principle will become apparent as the exchange of gases between blood and lungs, and between blood and tissues, is examined more closely.
Gas exchange at the lungs
The site of gas exchange between blood and lungs is the alveolar membrane, the thin lining of the microscopic cul de sacs of lung structure, called alveoli. The millions of alveoli provide a massive alveolar membrane surface area for gas exchange: 80 m2 in the adult lung. On one side of the membrane is alveolar air. On the other are blood capillaries with diameter so small that red blood cells can only pass through in essentially ‘single file’. Gases diffuse across this membrane in an attempt to equalise the partial pressure (amount) of each gas on either side of the membrane. So that oxygen diffuses from alveoli (PO2 13.3 kPa) to blood (PO2 5.3 kPa) and carbon dioxide diffuses from the blood (PCO2 6.1 kPa) to alveoli (PCO2 5.3 kPa).
Adequate gas exchange between the lungs and blood is dependent on:
- Adequate alveolar ventilation by lungs. This is the mechanical process, due to the elastic recoil of lungs, that ensures movement of air in and out of alveoli.
- Normal numbers of functioning alveoli.
- Sufficient blood flow through the pulmonary capillaries (i.e. adequate perfusion of alveoli).
Transport of oxygen in blood
Oxygen passes across the alveolar membrane into the blood flowing through pulmonary capillaries. Oxygen is poorly soluble in blood and the small amount of oxygen that can be transported simply dissolved in blood (around 3.0 ml of oxygen per litre of blood) is quite inadequate to satisfy the body’s demand for oxygen. The oxygen carrying protein, haemoglobin, contained in red blood cells (erythrocytes) provides an additional, far more effective, means of transporting oxygen that increases the oxygen carrying capacity of blood from 3.0 to 200 ml oxygen per litre. In fact only 1–2% of the oxygen transported in blood is dissolved in the blood; this small fraction determines the measured partial pressure of oxygen (PO2). The remaining 98–99% is transported in erythrocytes bound to haemoglobin.
The product of the reversible binding of oxygen by haemoglobin is called oxyhaemoglobin; the term deoxyhaemoglobin is used to describe haemoglobin that has less oxygen bound to it than is maximally possible. The oxygen delivery function of haemoglobin, that is its ability to ‘pick up’ oxygen in the lungs and ‘release’ it in the microvasculature of tissues, is made possible by a reversible conformational change in the quaternary structure (shape) of the haemoglobin molecule that alters its affinity for oxygen. In the deoxy state haemoglobin has low affinity for oxygen and in the oxy state it has high affinity for oxygen. A number of environmental factors in blood determine the haemoglobin state (deoxy or oxy) and thereby relative affinity for oxygen. The most significant of these is the PO2. Haemoglobin present in blood with relatively high PO2 (arterial blood) has much greater affinity for oxygen than haemoglobin present in blood with relatively low PO2 (venous blood). The oxygen dissociation curve (ODC) describes this relationship graphically (Figure 7.2).
The percentage of total haemoglobin saturated with oxygen (i.e. oxygen saturation, SO2) is the measure of haemoglobin affinity in the graph in Figure 7.2. It is clear from the graph that at the high PO2 that prevails in the blood exposed to alveolar air in the lung (~12 kPa), haemoglobin is almost 100% saturated with oxygen; nearly all of the available oxygen binding sites on the totality of haemoglobin molecules are occupied with oxygen. By contrast in the milieu of the tissues where PO2 is much lower, haemoglobin affinity for oxygen is also much lower and oxygen is released from haemoglobin to the tissues.
Although arterial PO2 only reflects a tiny proportion (1–2%) of the oxygen in arterial blood, it is highly significant because it determines the amount of oxygen bound to haemoglobin (the SO2) and therefore the total amount of oxygen that is contained in arterial blood for delivery to tissues. If PO2 of arterial blood is reduced then less oxygen can be carried by haemoglobin (i.e. SO2 is reduced) and less oxygen is available to tissues. Examination of Figure 7.2 reveals that a significant decrease in PO2 from 16 kPa to 10 kPa has only slight effect on SO2 and therefore the oxygen content of blood, but there is a sharp fall in SO2 as PO2 falls below 10 kPa. The delivery of oxygen to tissues becomes increasingly compromised as PO2 of arterial blood falls below this level.
For adequate oxygenation of tissues then:
- Blood must contain sufficient haemoglobin.
- That haemoglobin must be >95% saturated with oxygen in arterial blood (SO2 >95%).
- To achieve >95% oxygen saturation, arterial blood PO2 must be >10 kPa (Figure 7.2).
- Maintenance of arterial PO2 above 10 kPa is dependent on the factors required for normal gas exchange between lungs and blood (already discussed).
Figure 7.2 Oxygen dissociation curve. Relationship between the amount of oxygen in blood PO2 and the amount of oxygen carried by haemoglobin (% Hb saturation).
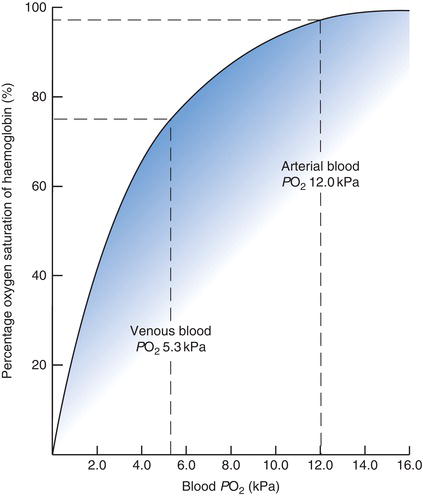
Acid-base balance: the maintenance of normal blood pH
Normal cellular metabolism requires that blood pH be maintained within the range 7.35–7.45 despite continuous production of hydrogen ions, which tend to reduce pH. Even slight excursions outside this range have deleterious effects and a pH of less than 6.8 or greater than 7.8 is considered incompatible with life. A brief review of some basic concepts is required for an understanding of acid-base balance in the body.
What is pH?
pH is a logarithmic scale (0–14) of acidity and alkalinity. Pure water has a pH of 7 and by convention neutral (i.e. neither acidic nor alkaline) pH above 7 is alkaline and pH less than 7 is acidic. The term pH is an abbreviation of puissance hydrogen (puissance is French for power). It is thus a measure of hydrogen ion activity or concentration. pH is defined as the negative log to the base 10 (i.e. log10) of the hydrogen ion concentration in mol/L or:
Eqn. 1
where [H+] = hydrogen ion concentration in mol/L
From this equation:
pH 7.4 = H+ concentration of 40 nmol/L
pH 7.0 = H+ concentration of 100 nmol/L
pH 6.0 = H+ concentration of 1000 nmol/L
It is evident that:
- The two parameters change inversely; as hydrogen ion concentration increases, pH falls.
- Due to the logarithmic nature of the pH scale, an apparently small change in pH is in fact a large change in hydrogen ion concentration. For example, a reduction of only 0.3 pH unit from 7.4 to 7.1 means a doubling of hydrogen ion concentration from 40 to 80 nmol/L.
Some laboratories report hydrogen ion concentration (units – nmol/L) in preference to pH.
What is an acid and a base?
An acid is a substance that dissociates in solution to release hydrogen ions. A base accepts hydrogen ions, for example, hydrochloric acid (HCl) dissociates to hydrogen ions and chlorine ions:
Eqn. 2
whereas bicarbonate (), a base, accepts hydrogen ions to form carbonic acid:
A strong acid like hydrochloric acid dissociates easily, yielding many hydrogen ions; it has therefore a very low pH.
A weak acid by contrast dissociates less easily yielding less hydrogen ions and therefore a relatively higher pH than a strong acid.
What is a buffer?
Chemical buffers are compounds in solution that resist change in pH caused by addition of an acid, by ‘mopping up’ hydrogen ions resulting from acid dissociation.
A buffer is the conjugate base of any weak acid. Because of its prime physiological importance for the maintenance of blood pH, the bicarbonate buffer system will be used as an example (there are several other buffer systems in blood). The buffer in this instance is bicarbonate, the conjugate base of the weak acid, carbonic acid. When a strong acid, for example, hydrochloric acid, is added to a solution of sodium bicarbonate (the buffer), the hydrogen ions from the strongly dissociating hydrochloric acid are incorporated into carbonic acid, a weakly dissociating acid:
Eqn. 4
The important point here is that because the hydrogen ions from hydrochloric acid have been incorporated into a weak acid, which does not dissociate readily, the total number of hydrogen ions in solution and therefore the pH does not change as much as would have occurred in the absence of the buffer. Although a buffer minimises changes in pH due to addition of hydrogen ions, it cannot entirely eliminate them because even weak acids dissociate to some extent. A very useful (if at first sight daunting!) equation defines the pH of all buffer systems in terms of the concentrations of their weak acid and conjugate base, it is called the Henderson-Hasselbach equation. For the bicarbonate buffer system then, this equation is:
Eqn. 5
Where [HCO3] is the concentration of the conjugate base, bicarbonate and [H2CO3] is the concentration of the weak acid, carbonic acid.
This equation reveals that pH is governed by the ratio of the concentration of base () to concentration of acid (H2CO3).
As hydrogen ions are added to bicarbonate (the buffer), the concentration of bicarbonate falls (as it is converted to carbonic acid) and the concentration of carbonic acid rises (Eqn.3). If acid (hydrogen ions) continues to be added to the system bicarbonate would eventually be consumed (all would be converted to carbonic acid). At this point there would be no buffering capacity and pH would fall sharply with addition of more acid. However if carbonic acid could be continuously removed from the system as it was generated, and bicarbonate continuously replenished, then buffering capacity and therefore pH could be maintained, despite continued addition of hydrogen ions.
As will become clear with more detail of the physiology of acid-base balance that is, in effect, what happens in the body. In essence the lungs ensure removal of carbonic acid (as carbon dioxide) and the kidneys ensure continuous regeneration of bicarbonate. The role of the lungs in maintenance of normal blood pH thus depends on a singular characteristic of the bicarbonate buffering system, the conversion of carbonic acid to carbon dioxide and water. The following equation outlines the relationship of all elements of the bicarbonate buffering system as it operates in the body:
It is important to note that the reactions are reversible. Direction is dependent on the relative concentration of each element. For example, a rise in carbon dioxide forces reaction to the left with increased production of carbonic acid and ultimately hydrogen ions. This explains the acidic potential of carbon dioxide and brings us to the important contribution that the lungs play in preserving normal blood pH.
Lungs and maintenance of normal blood pH
The main contribution of the lungs to the maintenance of a normal pH is regulation of the amount of carbon dioxide in blood. The actual amount of carbon dioxide in blood reflects a balance between that produced by cellular metabolism and that eliminated by the lungs in expired air, during respiration. Respiratory chemoreceptors in the brain detect changes in carbon dioxide content of blood, increasing respiration if carbon dioxide is high and reducing respiratory rate if low. Thus respiratory rate is the main determinant of carbon dioxide excretion by the lungs and therefore the amount of carbon dioxide in blood.
The sequence of events from CO2 production in the tissues to elimination in expired air is described in Figure 7.3.
Carbon dioxide diffuses out of tissue cells to surrounding capillary blood. A small proportion dissolves in blood plasma and is transported to the lungs unchanged. But most diffuses into red cells, where an enzyme called carbonic anhydrase facilitates its combination with water to form carbonic acid. The acid dissociates, with production of hydrogen ions and bicarbonate. Hydrogen ions combine with deoxygenated haemoglobin (haemoglobin is acting as a buffer here) preventing a dangerous fall in cellular pH, and bicarbonate diffuses along a concentration gradient from red cell to plasma. Thus most of the carbon dioxide produced in the tissues is transported to the lungs as bicarbonate in blood plasma. A small proportion of the carbon dioxide that diffuses into red cells is transported bound to haemoglobin.
At the alveoli in the lungs the process is reversed (Figure 7.3b). Hydrogen ions are displaced from haemoglobin as it takes up oxygen from inspired air. The hydrogen ions are now buffered by bicarbonate which diffuses from plasma back into red cell, and carbonic acid is formed. As the concentration of this rises it is converted to water and carbon dioxide. Finally carbon dioxide diffuses down a concentration gradient from red cell to alveoli for excretion in expired air.
Kidneys and maintenance of normal blood pH
Normal cellular metabolism results in continuous production of hydrogen ions. We have seen that by combining with these hydrogen ions, buffers in blood minimise their effect on pH. However buffering does not remove hydrogen ions from the body and maintenance of normal blood pH depends ultimately on the ability of the body to eliminate hydrogen ions. At the same time, it is important to continuously replenish the bicarbonate used in buffering. These two tasks: elimination of hydrogen ions and regeneration of bicarbonate are accomplished by the kidneys; specifically the renal tubule cells. These cells are rich in the enzyme carbonic anhydrase, which facilitates the formation of carbonic acid from carbon dioxide and water. The carbonic acid dissociates to hydrogen ions and bicarbonate. The bicarbonate is reabsorbed to blood and hydrogen ions pass into the lumen of the tubule and eliminated from the body in urine. This urine elimination of hydrogen ions depends on the presence in urine of buffers, principally phosphate and ammonia ions.
Figure 7.3 (a) Delivery of oxygen (O2) to tissues and first step in the elimination of carbon dioxide (CO2); (b) at the lung alveoli bicarbonate is converted back to carbon dioxide (CO2), which is eliminated by the lungs in expired air.
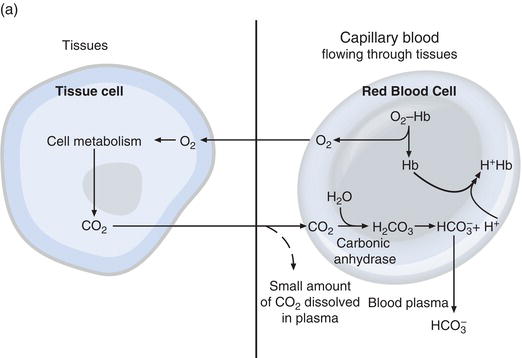
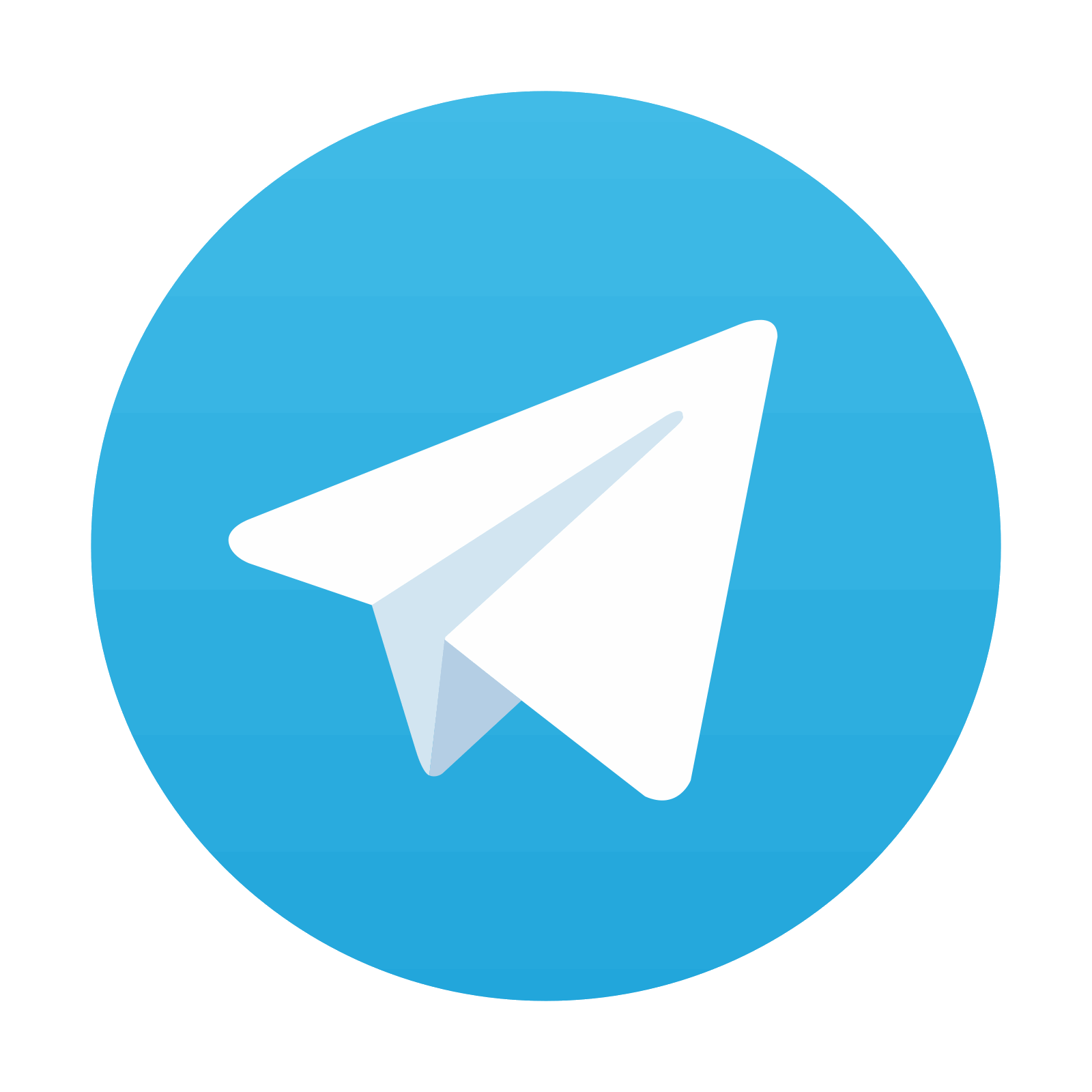
Stay updated, free articles. Join our Telegram channel
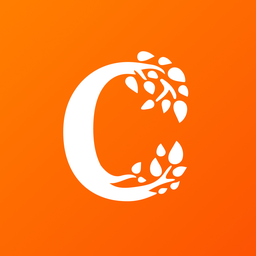
Full access? Get Clinical Tree
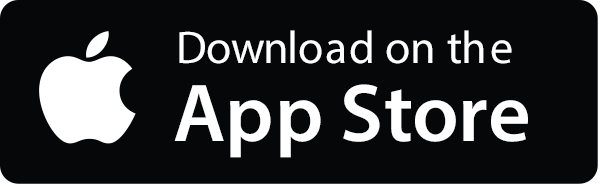
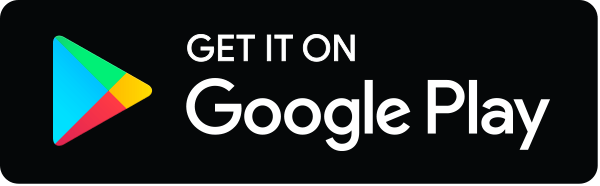