Regulation of Cardiac Output and Blood Pressure
Elizabeth J. Bridges
This chapter reviews the neurohumoral control of the cardiovascular system as it relates to the rapid and more long-term control of cardiac output and blood pressure and the local control of blood flow (autoregulatory, metabolic, autacoid). Several models of cardiac function are presented, including the relationship between cardiac output and central venous pressure, the Krogh model of the effect of distribution of blood volume on cardiac output, and the arterial baroreflex responses to decreased and increased blood pressure.

Arterial Baroreceptors
The arterial baroreceptors are responsible for the reflex control of blood pressure. These baroreceptors are undifferentiated nerve fibers located in the adventitia of the carotid sinus (at the bifurcation of the carotid artery) and the aortic arch (between the arch of the aorta and the bifurcation of the subclavian artery; Fig. 3-1). The receptors are mechanoreceptors that respond to distortion or a change in transmural pressure or stretch of the vascular bed in which they are located. For example, the carotid baroreceptors are sensitive to external compression or massage, both of which unload them (decrease transmural pressure). Although baroreceptors are often referred to as “pressoreceptors,” they in fact do not sense pressure directly, but instead only indirectly through change in stretch.
The baroreceptors respond to two types of input: static input (i.e., mean arterial pressure) and phasic input (i.e., pulsatile changes). Therefore, the baroreceptors are responsive to mean arterial pressure, pulse pressure, and the number of pulses per minute (e.g., heart rate).1 The static response has a threshold effect, that is, below a certain threshold of mean arterial pressure (20 to 50 mm Hg), the receptor stops firing. Above this threshold there is an increase in rate of receptor firing in proportion to the increase in mean pressure, until a plateau of the output is reached at saturation. The phasic response increases when the rate of change of pressure rises (increasing pressure) and decreases when the rate of change in pressure decreases.
Cardiopulmonary Receptors
Cardiopulmonary or low-pressure baroreceptors are located in the atria, ventricles, and pulmonary arteries and veins, with the cardiac baroreceptors providing the primary afferent input for the vagal cardioreflex.2,3 The properties of the cardiopulmonary baroreceptors are similar to those of the arterial baroreceptors, that is, a decrease in transmural pressure in the chamber or vessel results in a decrease in the firing rate of receptors, and vice versa.
Input to the central nervous system from the ventricular receptors, which are sensitive to mechanical and chemical stimuli, is through nonmyelinated vagal afferents (C fibers).4 In response to an increase in ventricular pressure, the mechanoreceptors were previously thought to stimulate a depressor response (decreased heart rate/vasodilation). The depressor reflex causes a decrease in heart rate, and may play a role in the alteration in vascular tone;5 although this response is less than the vascular response induced by increases in carotid or coronary arterial pressure. The ventricular mechanoreceptors appear to play a role only in protection from gross overdistention, possibly during myocardial ischemia.6,7 Chronic activation of cardiac receptors in heart failure cause increased activation of hypothalamic paraventricular neurons, which may contribute to resetting of the baroreceptor reflex and a sustained increase in sympathetic activation.8
Bezold-Jarisch Reflex
The Bezold-Jarisch reflex, which is the most commonly used model to explain the triggering of vasovagal (neurocardiogenic) syncope, is manifested as a triad of symptoms (bradycardia, apnea, and hypotension).9 Neurocardiogenic syncope is thought to occur as a result of excessive venous pooling and a decrease in peripheral venous return. The decreased venous return leads to a hypercontractile state that stimulates the cardiac mechanoreceptors (particularly those in the inferoposterior wall of the left ventricle).10 This hypercontractile state mimics hypertension and causes a paradoxical inhibitory or depressor reflex causing a vagally mediated decrease in heart rate and withdrawal of sympathetic stimulation to the peripheral vasculature with subsequent vasodilation.11 Stimulation of this reflex may occur with pathological conditions, such as myocardial infarction, administration of thrombolytic therapy, hemorrhage, aortic stenosis, or syncope. It is important to note that vasovagal syncope may also occur in patients with transplanted (denervated) hearts; thus, factors other than those traditionally attributed to the Bezold-Jarish reflex must be considered.9 Figure 3-2 characterizes the numerous putative causes of vasovagal syncope. During an acute inferoposterior myocardial infarction (particularly because of right coronary artery occlusion) and at the time of reperfusion of these infarctions, the transient bradycardia observed is thought to be a manifestation of the depressor effect of vagal receptors located in the inferoposterior wall of the left ventricle.12 Recent research also suggests that this response occurs with more proximal lesions involving the right ventricle.13 During ischemia, these receptors, which are mechanosensitive or chemosensitive, may be distorted by bulging of the ventricular wall during systole14 or by the presence of reactive oxygen species, serotonin, bradykinin, thromboxane A2, or adenosine.15, 16, 17 During thrombolytic therapy, the occurrence of vagally mediated bradycardia may be an indicator of reperfusion and sustained vessel patency, particularly with an inferior myocardial infarction.18 These receptors are also thought to mediate the reflex bradycardia and hypotension that occur during coronary angiography, particularly during injection of contrast material into the arteries that supply the inferoposterior surface of the left ventricle (e.g., circumflex, right coronary artery).19
In severe aortic stenosis, some patients experience exertional syncope and even sudden death. The probable mechanism of the syncope is an exercise-induced increase in left ventricular pressure, which is extreme because of high aortic valve resistance, despite a decrease in aortic blood pressure. This high left ventricular pressure stimulates the ventricular baroreceptors and is manifested by a Bezold-Jarisch response.20, 21, 22, 23 Once these patients undergo surgical correction of the stenosis, however, the normal sympathetic vasoconstrictor response to exercise is restored. Similarly, in patients with hypertrophic cardiomyopathy, this abnormal response may be the cause of syncope, exercise-induced paradoxical peripheral vasodilation, or sudden cardiac arrest.24, 25, 26 Finally, in cases of severe hemorrhage or during head-up tilt (particularly in patients receiving a concurrent infusion of isoproterenol), the ventricular depressor reflex is thought to be initiated by the acute distortion of the ventricular mechanoreceptors by a forceful ventricular contraction on a relatively empty ventricle or simply forceful contraction alone.27 In a trauma model, inhibition of Bezold-Jarisch mediated bradycardia with β-adrenergic blockade may aid in resuscitation.28
Chemoreceptors
Peripheral chemoreceptors located in the carotid and aortic bodies are sensitive to decreased arterial PaO2 or an increase in PaCO2 or [H+], whereas central chemoreceptors, which are located in the medulla are sensitive to increased PaCO2.29 Stimulation of these receptors leads to hyperventilation and sympathetic activation, which causes vasoconstriction in most vascular beds, except the brain and heart. While an increase in blood pressure is an outcome of the chemoreflex, an increase in baroreceptor stimulation (i.e., increased arterial blood pressure) inhibits the chemoreflex response. Conversely, the chemoreflexes potentiate the baroreflexmediated vasoconstriction in response to decreased arterial blood pressure.30 In hypertension and sleep apnea, the peripheral chemoreflex response to hypoxemia is enhanced, with a resultant increase in sympathetic activation. Of clinical importance, there is a strong relationship between hypertension and sleep apnea (i.e., individuals with sleep apnea have a high prevalence of hypertension).31 In heart failure, both the peripheral and central chemoreflex responses may be enhanced, as manifested by increased sympathetic activation.32 This enhanced response may contribute to genesis of sleep apnea in these patients, which is associated with a poorer prognosis.33, 34, 35 (See Chapter 8 for discussion of the relationship between sleep apnea and cardiovascular disease.)

The nucleus tractus solitarius is an ovoid area located in the medulla that receives efferent input from cardiovascular, respiratory, and gastrointestinal sites (see Fig. 3-1). The nucleus tractus solitarius serves as the first relay station for reflexes (e.g., baroreceptor reflex, central and peripheral arterial chemoreceptors, and skeletal muscle receptors [ergoreceptors]) that control circulation and respiration.36 From the nucleus tractus solitarius, there are multiple projections to areas such as: (1) the ventrolateral medulla, which is responsible for sympathetic efferent activity; (2) the nucleus ambiguus or “cardioinhibitory center” of the medulla, which is the location of the cell bodies of the vagal parasympathetic nerves; and (3) the median preoptic nuclei, which affect the release of vasopressin. The output from the medulla depends on the perturbation of the system (i.e., an increase or decrease in blood pressure). From the central nervous system, the efferent arm of the rapid control of blood pressure operates through the autonomic nervous system. From the carotid sinus, afferent input to the nucleus tractus solitarius in the medulla is through the carotid sinus nerve (nerve of Hering), which joins the ninth cranial nerve (glossopharyngeal). The sensory input from the aortic arch is through the 10th cranial nerve (vagus). Through synaptic connections to areas located in caudal and rostral ventrolateral medulla and nucleus ambiguus, sympathetic and parasympathetic output, respectively, is modified by afferent feedback from the baroreceptors. Output from the lateral ventrolateral medulla, which is directly projected to spinal sympathetic outflow via the bulbospinal (or medullospinal) tract, is responsible for maintaining tonic sympathetic activity, and thus resting arterial blood pressure.37,38 In addition, baroreceptor signals are transmitted to the forebrain. Paraventricular nuclei in the forebrain play a role in the release of vasopressin in response to a sustained decrease in blood pressure and increased osmolarity (or hypernatremia) and influences the sympathoexcitatory vasomotor neurons in the medulla.39,40 The excitation or inhibition of the sympathetic and parasympathetic systems depends on the direction of the change in arterial blood pressure. An example of the reflex response (increased parasympathetic activity in the heart and sympathetic activity in the heart and vasculature) to increased blood pressure is summarized in Figure 3-3.41 Of clinical importance, the baroreceptor reflex is reset at a higher point in hypertension, which is associated with adrenergic overdrive, decreased ability of cardiopulmonary receptors to control renin release and altered control of blood pressure and blood volume.42

The autonomic nervous system, which is one branch of the peripheral nervous system, is responsible for coordination of body functions that ensure homeostasis. The autonomic nervous system is further divided into two major components: the sympathetic nervous system and the parasympathetic nervous system (Fig. 3-4).
Sympathetic Nervous System
Efferent projections from the hypothalamus and medulla terminate in the intermediolateral cells located in the gray matter of the thoracic and lumbar (thoracolumbar) sections of the spinal column (specifically, T-1 to L-2). Hence, the sympathetic nervous system is often referred to as the thoracolumbar division of the autonomic nervous system. The neuronal cell bodies, which are located in the spinal column, are generally the origin of short preganglionic efferent fibers that innervate postsynaptic sympathetic neurons located in three general groupings of ganglia (a group of nerve cell bodies). The paravertebral ganglia are located in a bilateral chain-like structure adjacent to the spinal column. This chain extends from the superior cervical ganglia, located at the level of the bifurcation of the carotid artery, to ganglia located in the
sacral region. The prevertebral ganglia, which lie midline and anterior to the aorta and vertebral column, include the celiac, aorticorenal, and superior and inferior mesenteric ganglia. The third group of ganglia comprises the previsceral or terminal ganglia, which are located close to the target organs of the sympathetic nervous system. The previsceral ganglia have long preganglionic fibers and short postganglionic fibers. In contrast, the paravertebral and prevertebral ganglia give rise to long postganglionic fibers, which extend to the target organs of the sympathetic nervous system (e.g., heart, lungs, vascular smooth muscle, liver, kidneys, bladder, and reproductive organs; see Fig. 3-4). Of particular importance to the control of blood pressure are the sympathetic receptors located in the heart, vasculature, kidneys, and renal medulla.
sacral region. The prevertebral ganglia, which lie midline and anterior to the aorta and vertebral column, include the celiac, aorticorenal, and superior and inferior mesenteric ganglia. The third group of ganglia comprises the previsceral or terminal ganglia, which are located close to the target organs of the sympathetic nervous system. The previsceral ganglia have long preganglionic fibers and short postganglionic fibers. In contrast, the paravertebral and prevertebral ganglia give rise to long postganglionic fibers, which extend to the target organs of the sympathetic nervous system (e.g., heart, lungs, vascular smooth muscle, liver, kidneys, bladder, and reproductive organs; see Fig. 3-4). Of particular importance to the control of blood pressure are the sympathetic receptors located in the heart, vasculature, kidneys, and renal medulla.
Adrenoreceptors
At the target organs, the postganglionic fibers terminate at the neuroeffector junction and are separated from the adrenergic receptors (adrenoreceptors) by only a small junctional gap or cleft. The adrenoreceptors have been classified into two general groups: α-adrenergic receptors and β-adrenergic receptors. The receptor groups are further divided into general subtypes, β1, β2, and β3 and α1 and α2 (Table 3-1).43,44 Based on molecular cloning techniques, the α-receptors are further subdivided, with the α1 subclassified as (α1A, α1B, α1D).45 The α1-adrenergic receptors, which are now characterized as subtypes α1A, α1B, and α1D, are located in arteries, arterioles, and cutaneous and visceral veins. The α1A receptors are responsible for vessel contraction. The α1B receptors are thought to contribute to the maintenance of basal vascular tone and arterial blood pressure in conscious animals and are sensitive to exogenous agonists. Finally, the α1D receptors also play a role in vascular contraction, although they have a lesser effect than the α1B receptors.46 The α2 receptor has also been subclassified (α2A, α2B, and α2C). The α2 receptors, which have presynaptic and postsynaptic functions, are characterized as α2A/D, α2B, and α2C. The α2A/D and α2B receptors are present in large arteries but are located with greater density on the terminal arterioles, which act as precapillary sphincters to control the number of open capillaries and total capillary blood flow. The α2A/D receptors play the primary role in vasoconstriction.47,48 The α2B receptors also play a role in vasoconstriction and may contribute to the onset of hypertension. The α2C receptors are responsible for venoconstriction.46,49
Table 3-1 ▪ CARDIOVASCULAR EFFECTS OF AUTONOMIC NERVOUS SYSTEM INNERVATION | ||||||||||||||||||||||||||||||||||||||||
---|---|---|---|---|---|---|---|---|---|---|---|---|---|---|---|---|---|---|---|---|---|---|---|---|---|---|---|---|---|---|---|---|---|---|---|---|---|---|---|---|
|
The effects of dopamine are mediated by two families of receptors (D1 and D2). The D1-like receptors (D1 and D5 receptor subtypes) couple with G proteins to activate adenyl cyclase and the D2-like receptors (D2, D3, and D4 receptor subtypes) inhibit adenyl cyclase release and activate potassium channels.50,51 Dopamine is a precursor of norepinephrine. In the heart, dopamine exerts its indirect inotropic and chronotropic effects through the release of norepinephrine. Stimulation of postjunctional D1 receptors in the renal, mesenteric, and splenic arteries produces vasodilation and natriuresis. Defects in the D1 and D5 receptor may be associated with the development of hypertension.50,52 Stimulation of prejunctional D2 receptors in blood vessels inhibits norepinephrine release causing vasodilation. Additionally, in the kidneys stimulation of the D2 receptor inhibits norepinephrine release and plays a synergistic role in modulating natriuresis via inhibition of aldosterone secretion.52, 53, 54, 55 Exogenous administration of low-dose dopamine (<4 μg/kg per minute) causes vasodilation of the renal and splanchnic vascular beds and increases sodium excretion. However, low-dose dopamine is not reno-protective.56, 57, 58 Intermediate doses of exogenous dopamine (2 to 10 μg/kg per minute) stimulate β1-adrenergic receptors in the heart and increases contractility. Higher doses (>10 μg/kg per minute) stimulate α-adrenergic receptors in the peripheral vasculature and cause vasoconstriction.
Heart.
In the heart, β1 receptors predominate (80%), although there are also a smaller number of β2 receptors (20%), with the β2 receptors playing a role in coronary vasodilation.59 Stimulation of the β1 and β2 receptors in the heart increases: (1) the rate of discharge of the sinoatrial node, (2) conduction across the atrioventricular node, and (3) speed of contraction in the atria and ventricles (chronotropic effect). In addition, β1 stimulation increases cardiac contractility (inotropic effect). There is also a small number (<1%) of β3 adrenergic receptors in cardiomyocytes.60 The β3 receptors, which mediate negative inotropy via a nitric oxide-dependent pathway,61 become important during heart failure when they are upregulated and while protective may contribute to functional degradation of the failing heart.60,62 There are small number (approximately 14%) of α1 receptors located in the atria and ventricles.63 Stimulation of the α1 receptors creates a modest inotropic response.64
Vasculature.
Sympathetic stimulation of the arterial tree extends to the level of the terminal arterioles and is also present on capacitance vessels, primarily in the splanchnic bed. The primary transmitter of the vascular smooth muscle sympathetic neuroeffector junction is norepinephrine. Binding of norepinephrine to the vascular smooth muscle α1 receptor initiates vasoconstriction. The distribution of the α1 subtypes varies depending on the vascular bed. For example, α1A adrenoreceptors predominate in coronary, splanchnic, renal, and pulmonary vessels, whereas central arteries and veins express all three α1 receptor subtypes.46,65 Stimulation of presynaptic α2 receptors inhibits norepinephrine release and decreases vasoconstriction, a process called passive vasodilation. Conversely, stimulation of the postsynaptic α2 receptors, which are located on large arterioles and perhaps most importantly on the terminal arterioles, causes vasoconstriction.49 This vasoconstriction determines the number of open capillaries, and thus capillary blood flow. The α2-mediated vasoconstriction of the terminal arterioles can be inhibited by metabolic vasodilators (e.g., oxygen, potassium), particularly in the skeletal muscles. In vascular smooth muscle, the β-adrenergic receptors are predominantly of the β2-subtype. Stimulation of these receptors causes vasorelaxation.44
Cutaneous Vasculature
Control of the cutaneous circulation arises from both thermoregulatory and nonthermoregulatory reflexes. The cutaneous circulation has an extensive distribution of both α1 and α2 adrenoreceptors, but virtually no β adrenoreceptors.66 The glabrous skin (e.g., palms/soles) is innervated only by vasoconstrictive nerves. In contrast, nonglabrous skin receives both vasoconstrictive and vasodilator innervations.67 The sympathetic vasoconstrictor nerves release norepinephrine and may also release vasoconstrictive cotransmitters (neuropeptide Y [NPY] or adenosine triphosphate [ATP]), which augments vasoconstriction.68,69
In a thermoneutral environment, the cutaneous resistance vessels in the acral regions (e.g., ears) are tonically constricted, whereas the nonacral regions (limbs, head, and trunk) have minimal constriction.70,71 Vasodilation in the acral regions is primarily caused by withdrawal of vasoconstrictive tone (passive vasodilation), whereas vasodilation in nonacral regions is the result of an active process, which is sympathetically (but not adrenergically) mediated. Within a “neutral zone,” thermoregulation is controlled entirely by changes in cutaneous vasomotor tone.72 An active increase in adrenergic tone causes vasoconstriction in response to hypothermia. Conversely, a decrease in adrenergic stimulation causes passive vasodilation and is responsible
for 10% to 20% of vasodilation in response to hyperthermia.67,68,73 Cholinergic nerves, which innervate the sweat glands, release a yet to be described co-transmitter that may be functionally linked to the large and important active cutaneous vasodilation seen in heat stress.69,73,74 Additionally, under conditions of hyperthermia, nitric oxide is necessary for the vasodilatory response.69 Endothelial nitric oxide (eNOS) is responsible for vasodilation in response to local cutaneous heating,75 whereas neuronal nitric oxide (nNOS) is responsible for vasodilation in response to whole-body heating.76 The cutaneous veins constrict in response to local cold and are reflexly constricted in response a decrease in skin or core body temperature.77
for 10% to 20% of vasodilation in response to hyperthermia.67,68,73 Cholinergic nerves, which innervate the sweat glands, release a yet to be described co-transmitter that may be functionally linked to the large and important active cutaneous vasodilation seen in heat stress.69,73,74 Additionally, under conditions of hyperthermia, nitric oxide is necessary for the vasodilatory response.69 Endothelial nitric oxide (eNOS) is responsible for vasodilation in response to local cutaneous heating,75 whereas neuronal nitric oxide (nNOS) is responsible for vasodilation in response to whole-body heating.76 The cutaneous veins constrict in response to local cold and are reflexly constricted in response a decrease in skin or core body temperature.77
Nonthermoregulatory control of the cutaneous circulation via the arterial and cardiopulmonary baroreflexes plays a role in blood pressure control. For example, under normothermic conditions, “unloading” of the baroreflex causes cutaneous vasoconstriction. Because of the normally low cutaneous blood flow during normothermia, this vasoconstriction contributes little to blood pressure maintenance. However, under conditions of hyperthermia and during exercise, when there is significant blood flow to the cutaneous vasculature, baroreflex-mediated vasoconstrictive may offset thermoregulatory vasodilation and play an important role in maintenance of blood pressure.67,73 Of note, the baroreflex sensitivity is not impaired by whole-body heating as previously thought; however, heat stress may decrease peripheral vasoconstrictor responsiveness, which contributes to an increased susceptibility to orthostatic intolerance.78
Neurotransmitters
The sympathetic postganglionic fibers that innervate the arterial tree are in general noradrenergic (i.e., release norepinephrine). The only exceptions are the postganglionic fibers that innervate the sweat glands (sudomotor neurons), which have acetylcholine as their neurotransmitter and the extrapyramidal system, which has dopamine as the primary neurotransmitter. Norepinephrine is synthesized from tyrosine and is stored in sympathetic nerve terminals. In response to neuronal stimulation, the “packets” or quanta of norepinephrine are extruded from the axon vesicles by exocytosis. The vesicular release of norepinephrine is enhanced by angiotensin II and cold, whereas the prejunctional effects of potassium, decreased PO2, heat, autacoids (adenosine, bradykinin, serotonin, and prostaglandins), nitric oxide, and acetylcholine inhibit its release79 (Fig. 3-5). The neurotransmitters diffuse over varying small distances, depending on the width of the junctional cleft, to receptors located on effector organs. Norepinephrine is also considered a systemic hormone because of its spillover into the interstitial space.
Parasympathetic Nervous System
The second branch of the autonomic nervous system is the parasympathetic nervous system. The primary parasympathetic outflow is through four cranial nerves (III, VII, IX, and X). Of importance to blood pressure and cardiac output control, cardiac vagal (cranial nerve X) motorneurons are located in the nucleus ambiguus and dorsal vagal nucleus of the medulla. In addition, there are cell bodies located in the spinal cord gray matter at S-2 through S-4. Hence, the parasympathetic nervous system is referred to as the craniosacral branch of the autonomic nervous system. In contrast to the sympathetic nervous system, the preganglionic fibers of the parasympathetic nervous system are long fibers, synapsing on ganglia that are close to or directly attached to the effector organ. The postsynaptic fibers are relatively short, in contrast to the fibers of the sympathetic nervous system.
Receptors
In the parasympathetic nervous system, the nerve fibers are cholinergic, which means they liberate acetylcholine. Despite a common neurotransmitter (acetylcholine), stimulation of various receptors in the parasympathetic nervous system causes different effects. The reason for the variable response is that there are two general types of cholinergic receptors: nicotinic and muscarinic.
Preganglionic cholinergic receptors, which are found in the sympathetic and parasympathetic nervous systems, are nicotinic. The nicotinic receptors are located on autonomic ganglia and skeletal muscle endplates. Stimulation of the nicotinic receptors is excitatory and short-term (milliseconds). These receptors are blocked by curare. In clinical practice, blockade of the nicotinic receptors with various neuromuscular-blocking agents (e.g., succinylcholine, pancuronium) causes musculoskeletal paralysis (blockade at the skeletal muscle endplate) and may potentially cause hypotension because of blockade at the autonomic ganglia.80
The primary postganglionic receptor in the heart, smooth muscle, and glandular tissue is muscarinic. These receptors are stimulated by muscarine and can be antagonized by atropine and scopolamine. There are subtypes of the muscarinic receptors that result in varied responses. The primary muscarinic receptors in the heart are the muscarinic subtype 2 (M2), which are specifically associated with vagal nerve endings in the heart. The M2 receptors have direct and indirect negative inotropic and chronotropic
effects.81 The direct effects are secondary to occupation of the β-adrenergic receptors and inhibition of norepinephrine release, and the indirect effects occur through inhibition of the adrenergic second messenger cAMP.82,83 There are also M1, M3, and M5 receptors in the heart, which may have pharmacologic implications.84 Of clinical importance, the negative chronotropic and inotropic effects associated with the M2 receptor are blocked by atropine.
effects.81 The direct effects are secondary to occupation of the β-adrenergic receptors and inhibition of norepinephrine release, and the indirect effects occur through inhibition of the adrenergic second messenger cAMP.82,83 There are also M1, M3, and M5 receptors in the heart, which may have pharmacologic implications.84 Of clinical importance, the negative chronotropic and inotropic effects associated with the M2 receptor are blocked by atropine.
Co-transmitters
At the preganglionic synapse, the primary neurotransmitter for the sympathetic and parasympathetic nervous systems is acetylcholine. At the neuroeffector junction in the sympathetic nervous system, the primary neurotransmitters are norepinephrine and its precursor, dopamine, whereas the primary neurotransmitter of the postganglionic fibers of the parasympathetic nervous system is acetylcholine. However, other neurotransmitters that augment or modify the effects of the primary neurotransmitter are coreleased, and are referred to as co-transmitters (Fig. 3-6).85, 86, 87 The most prominent co-transmitters in the sympathetic nervous system ganglia are NPY and ATP.86,88 Vasoactive intestinal peptide (VIP) is the prominent co-transmitter in the parasympathetic nervous system ganglia and nonadrenergic, noncholinergic nerves.89
Neuropeptide Y
NPY is an amino acid peptide released with norepinephrine from sympathetic nerve terminals. NPY has direct pressor effects and also exerts a prejunctional modulation of the release of other neurotransmitters. For example, NPY inhibits the release of acetylcholine from vagal nerve endings, thus attenuating the effects of the parasympathetic system on heart rate, atrioventricular conduction, and atrial contractility.90,91 In addition, NPY potentiates the postjunctional contractile effects of norepinephrine. In the mesentery, 30% of the sympathetic nervous system induced vasoconstriction depends on NPY,92,93 although the role of NPY varies depending on the vascular bed. NPY is also associated with vascular remodeling (Y1 receptor) and angiogenesis (Y2). Pharmacologic strategies that promote angiogenesis but inhibit the pro-atherosclerotic effects of NPY may be useful in preventing or treating pathological vascular remodeling.94,95
Vasoactive Intestinal Peptide
VIP is present in the peripheral and central circulation, where it acts as a nonadrenergic, noncholinergic neurotransmitter, or neuromodulator. Endogenous VIP is a potent vasodilator, although its effects vary in different vascular beds. It is released in response to vagal stimulation in the heart, where it produces coronary vasodilation
(effect greater on the arteries than the veins) as well as positive inotropic (particularly in the right atria and ventricle) and chronotropic effects.96,97 VIP-induced peripheral vasodilation is caused by increased calcium-extrusion or sequestration induced by VIP or natriuretic protein-C receptor stimulation. This peripheral vasodilation enhances the VIP-mediated inotropic effects.98
(effect greater on the arteries than the veins) as well as positive inotropic (particularly in the right atria and ventricle) and chronotropic effects.96,97 VIP-induced peripheral vasodilation is caused by increased calcium-extrusion or sequestration induced by VIP or natriuretic protein-C receptor stimulation. This peripheral vasodilation enhances the VIP-mediated inotropic effects.98

In addition to the rapid control of arterial pressure by the autonomic nervous system, hormones such as epinephrine and arginine vasopressin (AVP) directly and indirectly affect the baroreceptor reflex and play an important role in the rapid control of blood pressure. Three interrelated systems (natriuretic peptide system, renin-angiotensin-aldosterone system [RAAS], and the kallikrein-kinin system [KKS]) also contribute to the regulation of the arterial blood pressure and fluid volume (Fig. 3-7). Finally, a spillover of norepinephrine into the systemic circulation also affects blood pressure and cardiac output.
Epinephrine
In response to physical or emotional stressors (mental stress, exercise, hyperthermia, hypoglycemia), epinephrine is secreted into the plasma by the adrenal medulla, causing the plasma level of epinephrine to increase. Epinephrine stimulates β1 receptors in the heart and has positive chronotropic and inotropic effects. The net effect of this cardiac stimulation is an increase in cardiac output. Epinephrine also acts on the vasculature and stimulates the β2 receptors in the skeletal muscles and splanchnic arterioles, which cause vasodilation in these two large regions and potentially large decrements in the systemic vascular resistance. In the skin and kidneys, epinephrine stimulates the α-adrenergic receptors and causes vasoconstriction.99
Exogenously administered epinephrine has dose-specific effects. Low-dose epinephrine (0.1 mcg/kg per minute) stimulates the β1 and β2 adrenoreceptors and causes vasodilation and increased heart rate and contractility. Increased doses (>0.2 mcg/kg per minute) stimulate the α-adrenoreceptors and increases vascular resistance and blood pressure.100 Knowledge of these dose-specific effects is important, and although epinephrine is often administered for its vasoconstrictive effects, it may cause vasodilation with a smaller dose.
Arginine Vasopressin
AVP, or antidiuretic hormone (ADH), is a neurotransmitter synthesized in the hypothalamus and released from the neurohypophysis of the pituitary gland (posterior pituitary gland). Vasopressin is primarily released in response to changes in plasma osmolality; however, AVP may also be released in response to a decrease in blood volume or blood pressure. As the osmolality increases, AVP secretion increases. In humans, the primary effect of AVP is its antidiuretic effect, which is caused by stimulation of water absorption at the distal and collecting tubules of the kidney.101,102 The change in water absorption affects plasma osmolality. Vasopressin is exquisitely sensitive to changes in osmolality; for example, a 5- to 10 mOsm increase in osmolality causes an increase in plasma AVP.103,104 The close relation between osmolality and AVP maintains plasma osmolality within 1% of normal under most conditions.105,106
The sensitivity of the baroreceptor system is less than that of the osmoreceptors, as demonstrated by the large (5% to 10%) iso-osmotic change in plasma volume required before vasopressin secretion is altered. However, during hemorrhage (plasma volume decreased by >5% to 10%), plasma levels of vasopressin are increased, in some cases 100-fold107 (Fig. 3-8). In this case, vasopressin acts in a manner similar to renin and norepinephrine, causing vasoconstriction and playing a supporting role to the sympathetic nervous system in the maintenance of blood pressure. The primary reflex controllers of the plasma volume-mediated release of vasopressin are the arterial baroreceptors and not the cardiac receptors.70,108 In hemorrhage, the change in intrathoracic volume alters the arterial systolic blood pressure, which decreases arterial baroreceptor stimulation, and leads to increased vasopressin secretion.109
Natriuretic Peptides
There are three natriuretic peptides (A, B, and C) that contribute to the regulation of blood pressure and electrolyte and volume homeostasis.110,111 Atrial natriuretic peptide (ANP)112 and brain natriuretic peptide (B-type natriuretic peptide [BNP]) are released from granules in the atria and ventricles in response to increased stretch (increased preload and afterload) and hormonal stimuli (e.g., angiotensin II, glucocorticoids, endothelin I, catecholamines).113,114 Of note, factors that stimulate BNP release may be different in a normal versus hypertrophied ventricle and after myocardial infarction, with hypoxia acting independent of ventricular dilation to stimulate BNP release.113 The actions of BNP are similar to ANP. C-type natriuretic peptide is widely distributed in the brain, kidneys, lungs, heart, and endothelial cells, and is released in response to shear stress. Dendroaspis natriuretic peptide has been recently discovered; however, its exact mechanism remains to be determined.
The heart has endocrine functions113 via the cardiac natriuretic peptides (ANP and BNP) that bind with natriuretic peptide receptors A and B (NPR-A and NPR-B) and cause diuresis and natriuresis. These actions subsequently decrease preload and blood pressure.115 Preload reduction may occur because of shifting of fluid from the intravascular to the extravascular space (increased vascular endothelial permeability) and possibly increased capillary hydrostatic pressure along with natriuresis.110,116 Additionally, ANP and BNP decrease sympathetic tone to the peripheral vasculature both centrally and peripherally, inhibit the RAAS by inhibiting angiotensin II-stimulated sodium and water transport in the proximal tubules, inhibit endothelin-1 production, improve ventricular relaxation, and lower the activation threshold for the vagal afferents, which suppresses the reflex tachycardia and vasoconstriction associated with the decrease in preload and cardiac output117,118 (Fig. 3-9). In severe heart failure, increased
levels of ANP may offset the detrimental effects of increased angiotensin-aldosterone and the sympathetic nervous system.111,119
levels of ANP may offset the detrimental effects of increased angiotensin-aldosterone and the sympathetic nervous system.111,119
Clinically, the short-term administration of intravenous BNP nesitiride (Natrecor) has been shown to improve hemodynamic function and decrease symptoms of acute decompensated heart failure compared with standard therapy120, 121, 122. However, meta-analyses indicate that there may be increased risk of worsening renal failure and increased 30-day mortality;123, 124, 125 thus caution must be taken when administering this medication.126
C-type natriuretic peptide, which is stored in endothelial cells, acts in a paracrine fashion and binds to natriuretic peptide receptor C (NPR-C), which is located in vascular smooth muscle. Note that other texts refer to binding to NPR-A.91 CNP couples to inhibitory G proteins (Gi) and causes inhibition of adenylate cyclase and activation of phospholipase-C leading to vasodilation. ANP also binds to NPR-C with similar inhibitory effects.117 Recent research suggests that CNP may be an endothelium-dependent hyperpolarizing factor, with actions in the peripheral and coronary vasculature117,127, 128, 129 (see Chapter 2). The peripheral vascular effect of CNP decreases venous return and subsequently decreases cardiac filling pressures, cardiac output, and arterial blood pressure. Unlike ANP and BNP, CNP has minimal renal actions.130 CNP is a potent coronary vasodilator and also has an antimitogenic effect on vascular smooth muscle, which may be protective against atheroma development and restenosis.131 Additionally, in an experimental model of myocardial infarction, CNP administration decreased the size of the infarct and myocardial dysfunction and protected against ischemic reperfusion injury, with possible mechanisms including CNP/NPR-C related coronary vasodilation and decreased heart rate.127
Renin-Angiotensin-Aldosterone System
The RAAS plays an important role in the long-term control of arterial blood pressure, regional blood flow, and sodium balance. The RAAS acts in a cascade fashion, initiated by the stimulation of renin release from the kidney. Renin is stored in and released from the juxtaglomerular cells near the renal afferent arterioles. Renin release is stimulated by three mechanisms. First, renin release occurs in response to increased sympathetic nervous system stimulation of the afferent and efferent arterioles in the renal glomeruli. The β-adrenergic receptors in the cells of the juxtaglomerular apparatus are sensitive to neurally released and systemic catecholamines. This neurally mediated response can be blocked by β-adrenergic blockers (e.g., propranolol). Second, renin release is stimulated by decreased renal perfusion pressure, distending the afferent arterioles (intrarenal baroreceptor pathway). Below a mean arterial pressure of 80 to 90 mm Hg, renin secretion is a steep and linear function of renal perfusion pressure. Finally, decreased sodium chloride concentration in the macula densa, which is located in the early distal tubule, stimulates the juxtaglomerular apparatus to secrete renin. Increased blood pressure decreases renin release by activating the baroreceptors causing a decrease in sympathetic tone, increasing pressure in the renal arterioles, and decreasing sodium chloride reabsorption in the proximal tubule, causing increased sodium chloride to reach the macula densa.
Angiotensin II is released through the proteolytic effects of renin on the plasma protein, angiotensinogen, which is synthesized and released into the plasma from the liver. Renin converts angiotensinogen to angiotensin I. Angiotensin I, which is inactive, is converted to angiotensin II by an angiotensin-converting enzyme (ACE) located in the plasma and vascular endothelium (primarily pulmonary).132 Pharmacologically, ACE inhibitors exert their effect at this level of the RAAS.133
Angiotensin II has two receptors (AT1 and AT2). The classic actions of angiotensin II, which are primarily mediated through AT1, include vasoconstriction and stimulation of aldosterone release. Angiotensin II causes vasoconstriction of the arterioles through a direct effect on the vascular smooth muscle and indirectly affects vascular tone by stimulating the formation of superoxide anions, which inhibit nitric oxide-mediated vasodilation, and by inducing endothelin-1 formation to cause further vasoconstriction.134, 135, 136 Angiotensin receptor blockers work primarily on the AT1 receptors.
The renal and splanchnic circulations are particularly sensitive to angiotensin II. Angiotensin II increases vascular resistance and stimulates the heart indirectly through its potentiating actions on the sympathetic nervous system. These effects include: (1) accelerating the synthesis and release of norepinephrine; (2) delaying neuronal reuptake of norepinephrine; (3) directly stimulating the sympathetic ganglia; and (4) facilitating the response to sympathetic activity and vasoconstrictor drugs.70
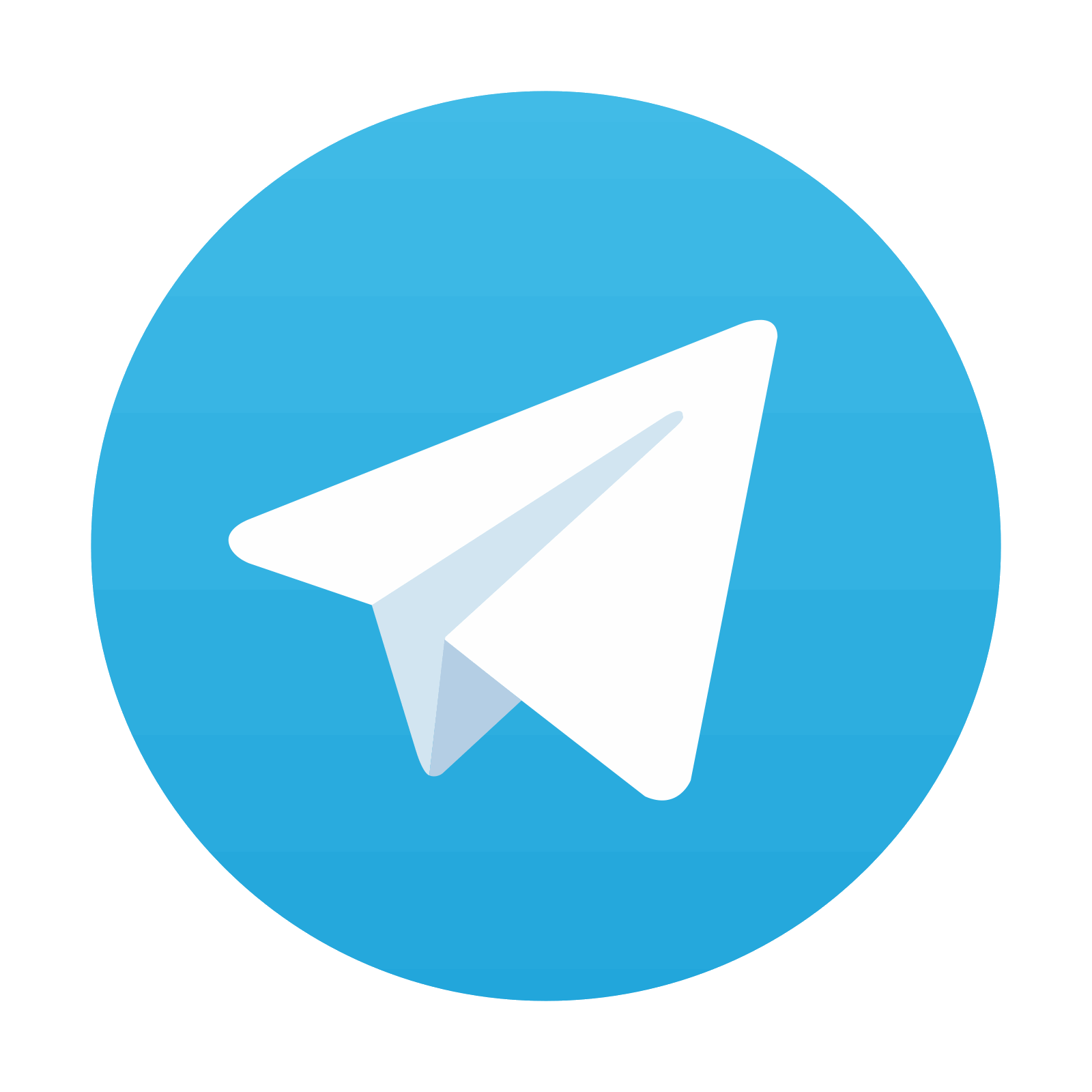
Stay updated, free articles. Join our Telegram channel
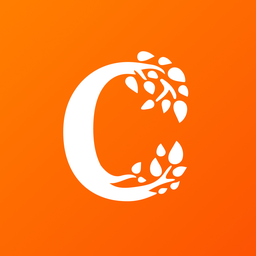
Full access? Get Clinical Tree
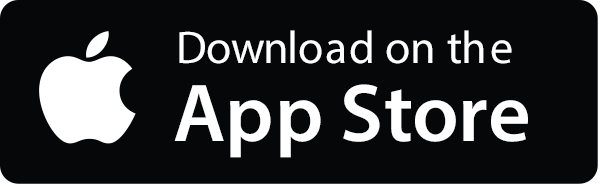
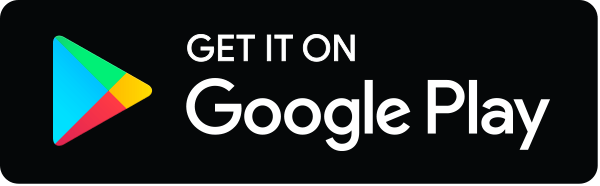